Discovery of the X-Ray Counterpart to the Gravitational Wave Event GW170817
Total Page:16
File Type:pdf, Size:1020Kb
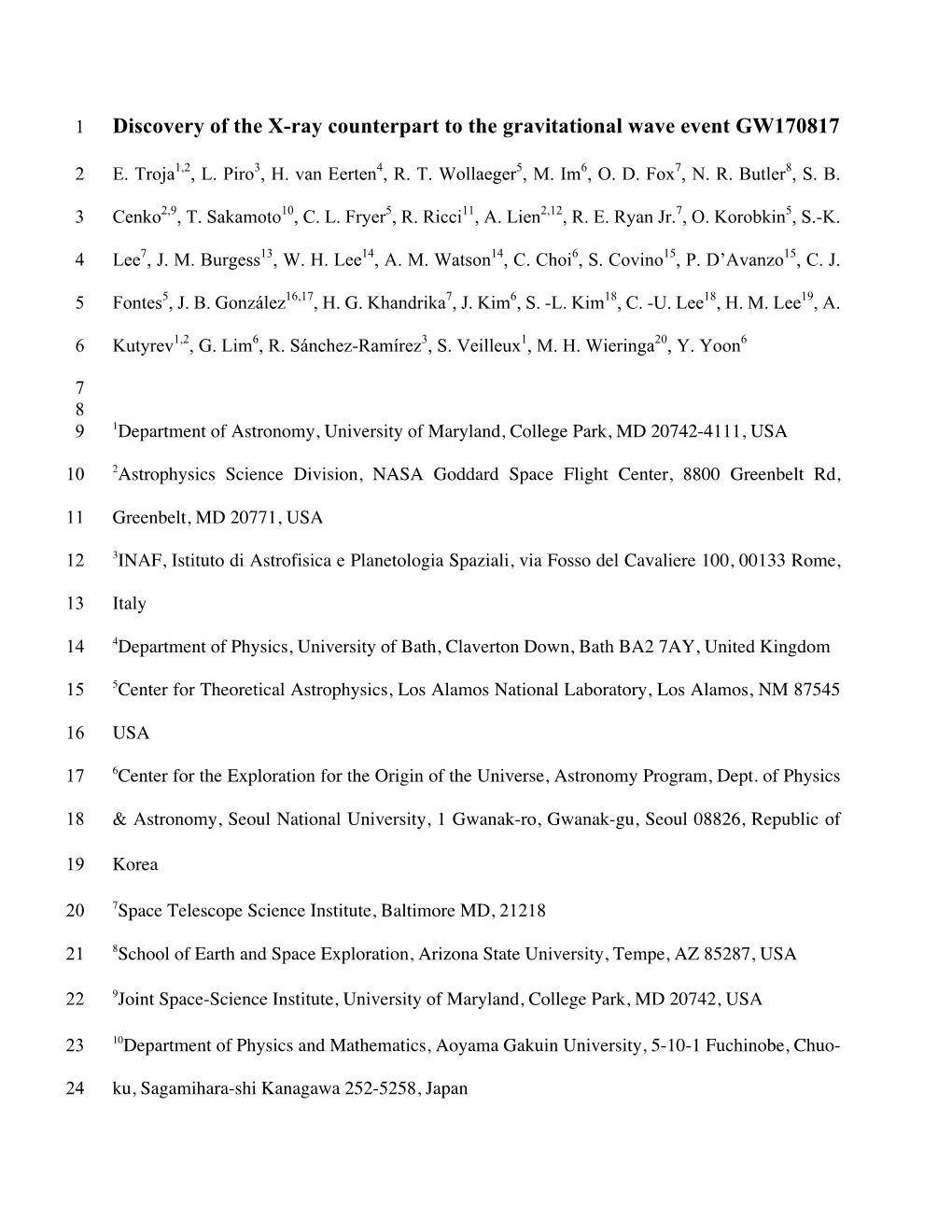
Load more
Recommended publications
-
Measurement of the Speed of Gravity
Measurement of the Speed of Gravity Yin Zhu Agriculture Department of Hubei Province, Wuhan, China Abstract From the Liénard-Wiechert potential in both the gravitational field and the electromagnetic field, it is shown that the speed of propagation of the gravitational field (waves) can be tested by comparing the measured speed of gravitational force with the measured speed of Coulomb force. PACS: 04.20.Cv; 04.30.Nk; 04.80.Cc Fomalont and Kopeikin [1] in 2002 claimed that to 20% accuracy they confirmed that the speed of gravity is equal to the speed of light in vacuum. Their work was immediately contradicted by Will [2] and other several physicists. [3-7] Fomalont and Kopeikin [1] accepted that their measurement is not sufficiently accurate to detect terms of order , which can experimentally distinguish Kopeikin interpretation from Will interpretation. Fomalont et al [8] reported their measurements in 2009 and claimed that these measurements are more accurate than the 2002 VLBA experiment [1], but did not point out whether the terms of order have been detected. Within the post-Newtonian framework, several metric theories have studied the radiation and propagation of gravitational waves. [9] For example, in the Rosen bi-metric theory, [10] the difference between the speed of gravity and the speed of light could be tested by comparing the arrival times of a gravitational wave and an electromagnetic wave from the same event: a supernova. Hulse and Taylor [11] showed the indirect evidence for gravitational radiation. However, the gravitational waves themselves have not yet been detected directly. [12] In electrodynamics the speed of electromagnetic waves appears in Maxwell equations as c = √휇0휀0, no such constant appears in any theory of gravity. -
Science Olympiad Astronomy C Division Event University of Chicago Invitational
Science Olympiad Astronomy C Division Event University of Chicago Invitational University of Chicago Chicago, IL January 11, 2020 Team Number: Team Name: Instructions: 1) Please turn in all materials at the end of the event. 2) Do not forget to put your team name and team number at the top of all answer pages. 3) Write all answers on the lines on the answer pages. Any marks elsewhere will not be scored. 4) Do not worry about significant figures. Use 3 or more in your answers, regardless of how many are in the question. 5) Please do not access the internet during the event. If you do so, your team will be disqualified. 6) Feel free to take apart the test and staple it back together at the end! 7) Good luck! And may the stars be with you! 1 Section A: Use the Image/Illustration Set to answer the following questions. Each sub-question in this section is worth one point. 1. Image 1 shows the Bullet Cluster. (a) What part of the electromagnetic spectrum was this image taken in? (b) What do the blue regions correspond to? (c) How was the matter in the blue regions detected? (d) Which other image shows this cluster? 2. Image 2 shows part of M87. (a) What part of M87 does this image show? (b) What part of the electromagnetic spectrum was this image taken in? (c) Which image shows a zoomed-in radio observation of this region? (d) What type of astronomical object is shown in the image from part (c)? 3. -
A Brief History of Gravitational Waves
universe Review A Brief History of Gravitational Waves Jorge L. Cervantes-Cota 1, Salvador Galindo-Uribarri 1 and George F. Smoot 2,3,4,* 1 Department of Physics, National Institute for Nuclear Research, Km 36.5 Carretera Mexico-Toluca, Ocoyoacac, C.P. 52750 Mexico, Mexico; [email protected] (J.L.C.-C.); [email protected] (S.G.-U.) 2 Helmut and Ana Pao Sohmen Professor at Large, Institute for Advanced Study, Hong Kong University of Science and Technology, Clear Water Bay, Kowloon, 999077 Hong Kong, China 3 Université Sorbonne Paris Cité, Laboratoire APC-PCCP, Université Paris Diderot, 10 rue Alice Domon et Leonie Duquet, 75205 Paris Cedex 13, France 4 Department of Physics and LBNL, University of California; MS Bldg 50-5505 LBNL, 1 Cyclotron Road Berkeley, 94720 CA, USA * Correspondence: [email protected]; Tel.:+1-510-486-5505 Academic Editors: Lorenzo Iorio and Elias C. Vagenas Received: 21 July 2016; Accepted: 2 September 2016; Published: 13 September 2016 Abstract: This review describes the discovery of gravitational waves. We recount the journey of predicting and finding those waves, since its beginning in the early twentieth century, their prediction by Einstein in 1916, theoretical and experimental blunders, efforts towards their detection, and finally the subsequent successful discovery. Keywords: gravitational waves; General Relativity; LIGO; Einstein; strong-field gravity; binary black holes 1. Introduction Einstein’s General Theory of Relativity, published in November 1915, led to the prediction of the existence of gravitational waves that would be so faint and their interaction with matter so weak that Einstein himself wondered if they could ever be discovered. -
Gravitational Waves and Gamma-Rays from a Binary Neutron Star Merger: Gw170817 and Grb 170817A
Draft version October 15, 2017 Typeset using LATEX twocolumn style in AASTeX61 GRAVITATIONAL WAVES AND GAMMA-RAYS FROM A BINARY NEUTRON STAR MERGER: GW170817 AND GRB 170817A B. P. Abbott,1 R. Abbott,1 T. D. Abbott,2 F. Acernese,3, 4 K. Ackley,5, 6 C. Adams,7 T. Adams,8 P. Addesso,9 R. X. Adhikari,1 V. B. Adya,10 C. Affeldt,10 M. Afrough,11 B. Agarwal,12 M. Agathos,13 K. Agatsuma,14 N. Aggarwal,15 O. D. Aguiar,16 L. Aiello,17, 18 A. Ain,19 P. Ajith,20 B. Allen,10, 21, 22 G. Allen,12 A. Allocca,23, 24 M. A. Aloy,25 P. A. Altin,26 A. Amato,27 A. Ananyeva,1 S. B. Anderson,1 W. G. Anderson,21 S. V. Angelova,28 S. Antier,29 S. Appert,1 K. Arai,1 M. C. Araya,1 J. S. Areeda,30 N. Arnaud,29, 31 K. G. Arun,32 S. Ascenzi,33, 34 G. Ashton,10 M. Ast,35 S. M. Aston,7 P. Astone,36 D. V. Atallah,37 P. Aufmuth,22 C. Aulbert,10 K. AultONeal,38 C. Austin,2 A. Avila-Alvarez,30 S. Babak,39 P. Bacon,40 M. K. M. Bader,14 S. Bae,41 P. T. Baker,42 F. Baldaccini,43, 44 G. Ballardin,31 S. W. Ballmer,45 S. Banagiri,46 J. C. Barayoga,1 S. E. Barclay,47 B. C. Barish,1 D. Barker,48 K. Barkett,49 F. Barone,3, 4 B. Barr,47 L. Barsotti,15 M. Barsuglia,40 D. Barta,50 J. -
Measuring the Hubble Constant with a Sample of Kilonovae
Measuring the Hubble Constant with a sample of kilonovae Michael W. Coughlin1;2,∗ Sarah Antier3, Tim Dietrich4;5, Ryan J. Foley6, Jack Heinzel7;8, Mattia Bulla9, Nelson Christensen7;8, David A. Coulter6, Lina Issa9;10, and Nandita Khetan11 1School of Physics and Astronomy, University of Minnesota, Minneapolis, Minnesota 55455, USA 2Division of Physics, Math, and Astronomy, California Institute of Technology, Pasadena, CA 91125, USA 3APC, UMR 7164, 10 rue Alice Domon et Leonie´ Duquet, 75205 Paris, France 4Institut fur¨ Physik und Astronomie, Universitat¨ Potsdam, Haus 28, Karl-Liebknecht-Str. 24/25, 14476, Potsdam, Germany 5Nikhef, Science Park 105, 1098 XG Amsterdam, The Netherlands 6Department of Astronomy and Astrophysics, University of California, Santa Cruz, CA 95064, USA 7Artemis, Universite´ Coteˆ d’Azur, Observatoire Coteˆ d’Azur, CNRS, CS 34229, F-06304 Nice Cedex 4, France 8Physics and Astronomy, Carleton College, Northfield, MN 55057, USA 9Nordita, KTH Royal Institute of Technology and Stockholm University, Roslagstullsbacken 23, arXiv:2008.07420v1 [astro-ph.HE] 17 Aug 2020 SE-106 91 Stockholm, Sweden 10Universite´ Paris-Saclay, ENS Paris-Saclay, Departement´ de Phyisque, 91190, Gif-sur-Yvette, France. ∗ Corresponding Author: [email protected]. 1 11Gran Sasso Science Institute (GSSI), I-67100 L’Aquila, Italy 1 Abstract Kilonovae produced by the coalescence of compact binaries with at least one neutron star are promising standard sirens for an independent measurement of the Hubble constant (H0). Through their detection via follow-up of gravitational-wave (GW), short gamma-ray bursts (sGRBs) or optical surveys, a large sample of kilonovae (even without GW data) can be used for H0 contraints. -
Gravitational Waves from Supernova Core Collapse Outline Max Planck Institute for Astrophysics, Garching, Germany
Gravitational Waves from Supernova Core Collapse Outline Max Planck Institute for Astrophysics, Garching, Germany Harald Dimmelmeier [email protected] Gravitational Waves from Supernova Core Collapse: What could the Signal tell us? Work done at the MPA in Garching Dimmelmeier, Font, M¨uller, Astron. Astrophys., 388, 917{935 (2002), astro-ph/0204288 Dimmelmeier, Font, M¨uller, Astron. Astrophys., 393, 523{542 (2002), astro-ph/0204289 Source Simulation Focus Session, Center for Gravitational Wave Physics, Penn State University, 2002 Gravitational Waves from Supernova Core Collapse Max Planck Institute for Astrophysics, Garching, Germany Motivation Physics of Core Collapse Supernovæ Physical model of core collapse supernova: Massive progenitor star (Mprogenitor 10 30M ) develops an iron core (Mcore 1:5M ). • ≈ − ≈ This approximate 4/3-polytrope becomes unstable and collapses (Tcollapse 100 ms). • ≈ During collapse, neutrinos are practically trapped and core contracts adiabatically. • At supernuclear density, hot proto-neutron star forms (EoS of matter stiffens bounce). • ) During bounce, gravitational waves are emitted; they are unimportant for collapse dynamics. • Hydrodynamic shock propagates from sonic sphere outward, but stalls at Rstall 300 km. • ≈ Collapse energy is released by emission of neutrinos (Tν 1 s). • ≈ Proto-neutron subsequently cools, possibly accretes matter, and shrinks to final neutron star. • Neutrinos deposit energy behind stalled shock and revive it (delayed explosion mechanism). • Shock wave propagates through -
Gravitational Waves and Gamma-Rays from a Binary Neutron Star Merger: Gw170817 and Grb 170817A
GRAVITATIONAL WAVES AND GAMMA-RAYS FROM A BINARY NEUTRON STAR MERGER: GW170817 AND GRB 170817A The gravitational-wave signal GW170817 was detected on August 17, 2017 by the Advanced LIGO and Virgo observatories. This is the first signal thought to be due to the merger of two neutron stars. Only 1.7 seconds after the gravitational-wave signal was detected, the Fermi Gamma-ray Burst Monitor (GBM) and the Anticoincidence Shield for the SPectrometer for the INTErnational Gamma-Ray Astrophysics Laboratory (INTEGRAL SPI-ACS) detected a short gamma-ray burst GRB 170817A. For decades astronomers suspected that short gamma-ray bursts were produced by the merger of two neutron stars or a neutron star and a black hole. The combination of GW170817 and GRB 170817A provides the first direct evidence that colliding neutron stars can indeed produce short gamma-ray bursts. INTRODUCTION FIGURES FROM THE PUBLICATION Gamma-Ray Bursts (GRBs) are some of the most energetic events For more information on the meaning of these figures, see the full publication here. observed in Nature. They typically release as much energy in just a few seconds as our Sun will throughout its 10 billion-year life. They occur approximately once a day and come from random points on the sky. These GRBs can last anywhere from fractions of seconds to thousands of seconds. However, we usually divide them in two groups based roughly on their duration, with the division being at the 2 second mark (although more sophisticated features are also taken into account in the classification). Long GRBs (>2 seconds) are caused by the core-collapse of rapidly rotating massive stars. -
R-Process Nucleosynthesis in Neutron Star Mergers and GW170817
r-Process nucleosynthesis in neutron star mergers and GW170817 Jonas Lippuner July 18, 2018 FRIB and the GW170817 Kilonova FRIB/NSCL/MSU, East Lansing MI Operated by Los Alamos National Security, LLC for the U.S. Department of Energy’s NNSA Los Alamos National Laboratory UNCLASSIFIED LA-UR-18-21867 Outline 1. r-Process nucleosynthesis overview 2. r-Process in neutron star mergers 3. Observational signature and first detection Los Alamos National Laboratory UNCLASSIFIED 3/19/2018 | 2 Solar system abundances Los Alamos National Laboratory UNCLASSIFIED 3/19/2018 | 3 Solar system abundances Los Alamos National Laboratory UNCLASSIFIED 3/19/2018 | 3 The s-process slow neutron capture 90Zr 91Zr 92Zr τ ≪ τ ∼ 2 − 5 89 β− n 10 10 yr Y 84Sr 86Sr 87Sr 88Sr 85Rb 87Rb 78Kr 80Kr 82Kr 83Kr 84Kr 86Kr 79Br 81Br 74Se 76Se 77Se 78Se 80Se 82Se 75As 70Ge 72Ge 73Ge 74Ge 76Ge 69Ga 71Ga 66Zn 67Zn 68Zn 70Zn 65Cu closed neutron shell Los Alamos National Laboratory UNCLASSIFIED 3/19/2018 | 4 The s-process slow neutron capture 90Zr 91Zr 92Zr τ ≪ τ ∼ 2 − 5 89 β− n 10 10 yr Y 84Sr 86Sr 87Sr 88Sr 85Rb 87Rb 78Kr 80Kr 82Kr 83Kr 84Kr 86Kr 79Br 81Br 74Se 76Se 77Se 78Se 80Se 82Se 75As 70Ge 72Ge 73Ge 74Ge 76Ge 69Ga 71Ga 66Zn 67Zn 68Zn 70Zn 65Cu closed neutron shell Los Alamos National Laboratory UNCLASSIFIED 3/19/2018 | 4 The r-process rapid neutron capture 90Zr 91Zr 92Zr τ ≪ τ ∼ n β− 10 ms – 10 s 89Y 84Sr 86Sr 87Sr 88Sr 85Rb 87Rb 78Kr 80Kr 82Kr 83Kr 84Kr 86Kr 79Br 81Br 74Se 76Se 77Se 78Se 80Se 82Se 75As 70Ge 72Ge 73Ge 74Ge 76Ge 69Ga 71Ga 66Zn 67Zn 68Zn 70Zn 65Cu neutron drip -
A Brief History of Gravitational Waves
Review A Brief History of Gravitational Waves Jorge L. Cervantes-Cota 1, Salvador Galindo-Uribarri 1 and George F. Smoot 2,3,4,* 1 Department of Physics, National Institute for Nuclear Research, Km 36.5 Carretera Mexico-Toluca, Ocoyoacac, Mexico State C.P.52750, Mexico; [email protected] (J.L.C.-C.); [email protected] (S.G.-U.) 2 Helmut and Ana Pao Sohmen Professor at Large, Institute for Advanced Study, Hong Kong University of Science and Technology, Clear Water Bay, 999077 Kowloon, Hong Kong, China. 3 Université Sorbonne Paris Cité, Laboratoire APC-PCCP, Université Paris Diderot, 10 rue Alice Domon et Leonie Duquet 75205 Paris Cedex 13, France. 4 Department of Physics and LBNL, University of California; MS Bldg 50-5505 LBNL, 1 Cyclotron Road Berkeley, CA 94720, USA. * Correspondence: [email protected]; Tel.:+1-510-486-5505 Abstract: This review describes the discovery of gravitational waves. We recount the journey of predicting and finding those waves, since its beginning in the early twentieth century, their prediction by Einstein in 1916, theoretical and experimental blunders, efforts towards their detection, and finally the subsequent successful discovery. Keywords: gravitational waves; General Relativity; LIGO; Einstein; strong-field gravity; binary black holes 1. Introduction Einstein’s General Theory of Relativity, published in November 1915, led to the prediction of the existence of gravitational waves that would be so faint and their interaction with matter so weak that Einstein himself wondered if they could ever be discovered. Even if they were detectable, Einstein also wondered if they would ever be useful enough for use in science. -
The Afterglow and Early-Type Host Galaxy of the Short GRB 150101B at Z = 0.1343
The Afterglow and Early-type Host Galaxy of the Short GRB 150101B at Z = 0.1343 The Harvard community has made this article openly available. Please share how this access benefits you. Your story matters Citation Fong, W., R. Margutti, R. Chornock, E. Berger, B. J. Shappee, A. J. Levan, N. R. Tanvir, et al. 2016. “The Afterglow and Early-type Host Galaxy of the Short GRB 150101B at Z = 0.1343.” The Astrophysical Journal 833, no. 2: 151. doi:10.3847/1538-4357/833/2/151. Published Version doi:10.3847/1538-4357/833/2/151 Citable link http://nrs.harvard.edu/urn-3:HUL.InstRepos:30510303 Terms of Use This article was downloaded from Harvard University’s DASH repository, and is made available under the terms and conditions applicable to Open Access Policy Articles, as set forth at http:// nrs.harvard.edu/urn-3:HUL.InstRepos:dash.current.terms-of- use#OAP DRAFT VERSION SEPTEMBER 1, 2016 Preprint typeset using LATEX style emulateapj v. 01/23/15 THE AFTERGLOW AND EARLY-TYPE HOST GALAXY OF THE SHORT GRB 150101B AT Z = 0:1343 ; ; ; W. FONG1 2 , R. MARGUTTI3 4 , R. CHORNOCK5 , E. BERGER6 , B. J. SHAPPEE7 8 , A. J. LEVAN9 , N. R. TANVIR10 , N. SMITH2 , ; P. A. MILNE2 , T. LASKAR11 12 , D. B. FOX13 , R. LUNNAN14 , P. K. BLANCHARD6 , J. HJORTH15 , K. WIERSEMA10 , A. J. VAN DER HORST16 , D. ZARITSKY2 Draft version September 1, 2016 ABSTRACT We present the discovery of the X-ray and optical afterglows of the short-duration GRB 150101B, pinpointing the event to an early-type host galaxy at z = 0:1343±0:0030. -
Gravitational Waves and Core-Collapse Supernovae
Gravitational waves and core-collapse supernovae G.S. Bisnovatyi-Kogan(1,2), S.G. Moiseenko(1) (1)Space Research Institute, Profsoyznaya str/ 84/32, Moscow 117997, Russia (2)National Research Nuclear University MEPhI, Kashirskoe shosse 32,115409 Moscow, Russia Abstract. A mechanism of formation of gravitational waves in 1. Introduction the Universe is considered for a nonspherical collapse of matter. Nonspherical collapse results are presented for a uniform spher- On February 11, 2016, LIGO (Laser Interferometric Gravita- oid of dust and a finite-entropy spheroid. Numerical simulation tional-wave Observatory) in the USA with great fanfare results on core-collapse supernova explosions are presented for announced the registration of a gravitational wave (GW) the neutrino and magneto-rotational models. These results are signal on September 14, 2015 [1]. A fantastic coincidence is used to estimate the dimensionless amplitude of the gravita- that the discovery was made exactly 100 years after the tional wave with a frequency m 1300 Hz, radiated during the prediction of GWs by Albert Einstein on the basis of his collapse of the rotating core of a pre-supernova with a mass of theory of General Relativity (GR) (the theory of space and 1:2 M (calculated by the authors in 2D). This estimate agrees time). A detailed discussion of the results of this experiment well with many other calculations (presented in this paper) that and related problems can be found in [2±6]. have been done in 2D and 3D settings and which rely on more Gravitational waves can be emitted by binary stars due to exact and sophisticated calculations of the gravitational wave their relative motion or by collapsing nonspherical bodies. -
Gravitational Wave Echoes from Black Hole Area Quantization
Prepared for submission to JCAP Gravitational wave echoes from black hole area quantization Vitor Cardoso,a;b Valentino F. Foit,c Matthew Klebanc aCentro de Astrofísica e Gravitação - CENTRA, Departamento de Física Instituto Superior Técnico - IST, Universidade de Lisboa, Lisboa, Portugal bTheoretical Physics Department, CERN 1 Esplanade des Particules, Geneva 23, CH-1211, Switzerland cCenter for Cosmology and Particle Physics New York University, New York, USA E-mail: [email protected], [email protected], [email protected] Abstract. Gravitational-wave astronomy has the potential to substantially advance our knowledge of the cosmos, from the most powerful astrophysical engines to the initial stages of our universe. Gravitational waves also carry information about the nature of black holes. Here we investigate the potential of gravitational-wave detectors to test a proposal by Beken- stein and Mukhanov that the area of black hole horizons is quantized in units of the Planck area. Our results indicate that this quantization could have a potentially observable effect on the classical gravitational wave signals received by detectors. In particular, we find distorted gravitational-wave “echoes” in the post-merger waveform describing the inspiral and merger of two black holes. These echoes have a specific frequency content that is characteristic of black hole horizon area quantization. arXiv:1902.10164v1 [hep-th] 26 Feb 2019 Contents 1 Introduction1 1.1 Quantization of black hole area1 1.2 Imprints on classical observables2 1.2.1 Gravitational-wave