Azimuthally-Differential Pion Femtoscopy Relative to the Econds and Third Harmonic in Pb-Pb Collisions Mohammad Saleh Wayne State University
Total Page:16
File Type:pdf, Size:1020Kb
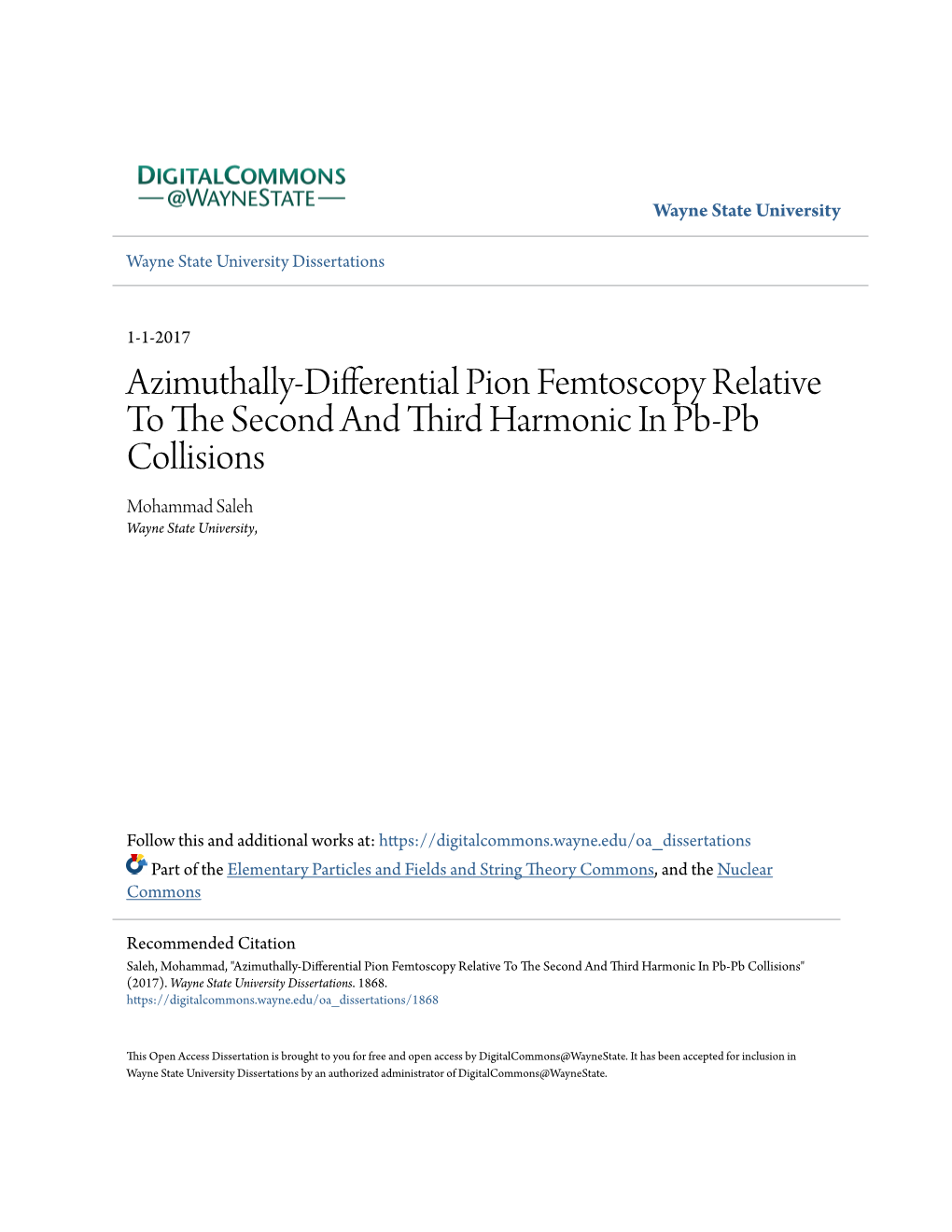
Load more
Recommended publications
-
Measurement of the J/ Elliptic Flow in Pb-Pb Collisions at Snn=5.02Tev
Measurement of the J/ elliptic flow in Pb-Pb collisions p at sNN=5.02TeV with the muon spectrometer of ALICE at the LHC Audrey Francisco To cite this version: p Audrey Francisco. Measurement of the J/ elliptic flow in Pb-Pb collisions at sNN=5.02TeV with the muon spectrometer of ALICE at the LHC. High Energy Physics - Experiment [hep-ex]. Ecole nationale supérieure Mines-Télécom Atlantique, 2018. English. NNT : 2018IMTA0087. tel-02010296 HAL Id: tel-02010296 https://tel.archives-ouvertes.fr/tel-02010296 Submitted on 7 Feb 2019 HAL is a multi-disciplinary open access L’archive ouverte pluridisciplinaire HAL, est archive for the deposit and dissemination of sci- destinée au dépôt et à la diffusion de documents entific research documents, whether they are pub- scientifiques de niveau recherche, publiés ou non, lished or not. The documents may come from émanant des établissements d’enseignement et de teaching and research institutions in France or recherche français ou étrangers, des laboratoires abroad, or from public or private research centers. publics ou privés. THESE DE DOCTORAT DE L’ÉCOLE NATIONALE SUPERIEURE MINES-TELECOM ATLANTIQUE BRETAGNE PAYS DE LA LOIRE - IMT ATLANTIQUE COMUE UNIVERSITE BRETAGNE LOIRE ECOLE DOCTORALE N° 596 Matière, Molécules, Matériaux Spécialité : Physique Subatomique et Instrumentation Nucléaire Par Audrey FRANCISCO Measurement of the J/� elliptic flow in Pb-Pb collisions at √sNN=5.02TeV with the muon spectrometer of ALICE at the LHC Thèse présentée et soutenue à Nantes le 24 Septembre 2018 Unité de recherche : SUBATECH— UMR6457 Thèse N° : 2018IMTA0087 Composition du Jury : Président : Pol-Bernard GOSSIAUX Professeur, IMT-Atlantique Examinateurs : Javier CASTILLO CASTELLANOS Chercheur-Ingénieur, CEA Manuel CALDERON DE LA BARÇA SANCHEZ Professeur, University of California Davis Marielle CHARTIER Professeur, University of Liverpool Silvia NICCOLAI Directeur de Recherche CNRS, Univ. -
Investigating Parton Energy Loss in the Quark-Gluon Plasma with Jet-Hadron Correlations and Jet Azimuthal Anisotropy at STAR
Investigating Parton Energy Loss in the Quark-Gluon Plasma with Jet-hadron Correlations and Jet Azimuthal Anisotropy at STAR A Dissertation Presented to the Faculty of the Graduate School of Yale University in Candidacy for the Degree of Doctor of Philosophy by Alice Elisabeth Ohlson Dissertation Director: John Harris December 2013 Copyright c 2013 by Alice Elisabeth Ohlson All rights reserved. ii Abstract Investigating Parton Energy Loss in the Quark-Gluon Plasma with Jet-hadron Correlations and Jet Azimuthal Anisotropy at STAR Alice Elisabeth Ohlson 2013 In high-energy collisions of gold nuclei at the Relativistic Heavy Ion Collider (RHIC) and of lead nuclei at the Large Hadron Collider (LHC), a new state of matter known as the Quark-Gluon Plasma (QGP) is formed. This strongly-coupled, deconfined state of quarks and gluons represents the high energy-density limit of quantum chro- modynamics. The QGP can be probed by high-momentum quarks and gluons (collec- tively known as partons) that are produced in hard scatterings early in the collision. The partons traverse the QGP and fragment into collimated “jets” of hadrons. Stud- ies of parton energy loss within the QGP, or medium-induced jet quenching, can lead to insights into the interactions between a colored probe (a parton) and the colored medium (the QGP). Two analyses of jet quenching in relativistic heavy ion collisions are presented here. In the jet-hadron analysis, the distributions of charged hadrons with respect to the axis of a reconstructed jet are investigated as a function of azimuthal angle and transverse momentum (pT). It is shown that jets that traverse the QGP are softer (consisting of fewer high-pT fragments and more low-pT constituents) than jets in p+p collisions. -
Anisotropic Flow Measurements in STAR at the Relativistic Heavy Ion Collider
Anisotropic Flow Measurements in STAR at the Relativistic Heavy Ion Collider Anisotrope stroming gemeten met de STAR detector aan de relativistische zware ionen versneller (met een samenvatting in het Nederlands) Proefschrift ter verkrijging van de graad van doctor aan de Universiteit Utrecht op gezag van de rector magnificus, prof. dr. J.C. Stoof, ingevolge het besluit van het college voor promoties in het openbaar te verdedigen op maandag 29 oktober 2007 des ochtends te 10:30 uur door Yuting Bai geboren op 31 december 1975 te Yulin, China Promotor: Prof. dr. Th. Peitzmann Co-promotor: Dr. R.J.M. Snellings Dit werk is een onderdeel van het onderzoeksprogramma van de “Stichting voor Fun- damenteel Onderzoek der Materie” (FOM), financieel gesteund door de “Nederlandse Organisatie voor Wetenschappelijk Onderzoek” (NWO). Contents 1 Introduction 5 1.1QuarkGluonPlasma.............................. 5 1.2 Heavy Ion Collisions .............................. 7 1.3Probes...................................... 8 1.3.1 Hard probes ............................... 8 1.3.2 Flow . .................................. 9 1.3.3 Anisotropic flow ............................ 12 1.4 Model description ................................ 14 1.4.1 Hydrodynamics ............................. 14 1.4.2 RQMD and UrQMD .......................... 16 1.4.3 AMPT .................................. 16 2 Experimental Set-up 17 2.1RHIC...................................... 17 2.2TheSTARDetector.............................. 22 2.2.1 STAR TPC ............................... 24 2.2.2 STAR FTPCs .............................. 27 2.2.3 Trigger ................................. 28 2.2.4 Centrality definition .......................... 29 dE 2.2.5 Particle Identification: dx ....................... 31 3 Flow analysis method 35 3.1 Two particle correlation ............................ 35 3.1.1 Event plane method .......................... 35 3.1.2 Scalar product method ......................... 37 3.2 Multiparticle correlation ............................ 38 3.2.1 Cumulant method .......................... -
Anisotropic Flow Measurements in ALICE at the Large Hadron Collider
Anisotropic Flow Measurements in ALICE at the Large Hadron Collider CERN-THESIS-2012-018 07/03/2012 A catalogue record is available from the Utrecht University Library. ISBN: Copyright c 2012 by A. Bilandzic All rights reserved. No part of this book may be reproduced, stored in a database or retrieval system, or published, in any form or in any way, electronically, mechanically, by print, photoprint, microfilm or any other means without prior written permission of the author. Typeset using LATEX and printed by Ipskamp Drukkers, Enschede, The Netherlands. Anisotropic Flow Measurements in ALICE at the Large Hadron Collider Anisotrope stroming gemeten met de ALICE detector aan de grote hadronen-botser (met een samenvatting in het Nederlands) Proefschrift ter verkrijging van de graad van doctor aan de Universiteit Utrecht op gezag van de rector magnificus, prof. dr. G.J. van der Zwaan, ingevolge het besluit van het college voor promoties in het openbaar te verdedigen op woensdag 7 maart 2012 des ochtends te 12.45 uur door Ante Bilandˇzi´c geboren te Split, Croatia Promotor: Prof. dr. R.J.M. Snellings Dit werk is een onderdeel van het onderzoeksprogramma van de “Stichting voor Fun- damenteel Onderzoek der Materie” (FOM), financieel gesteund door de “Nederlandse Organisatie voor Wetenschappelijk Onderzoek” (NWO), Grant number 680-47-217. It’s all like an ocean, I tell you. F.M.D. Contents 1 Introduction 11 1.1 Quantum Chromodynamics (QCD) . 11 1.2 Quark-gluon Plasma (QGP) . 12 1.3 Hydrodynamics and anisotropic flow . 14 1.3.1 Introduction to hydrodynamics in the relativistic heavy-ion collisions 14 1.3.2 Formalism of the relativistic ideal and viscous hydrodynamics . -
Letter of Intent for J-PARC Heavy-Ion Program (J-PARC-HI)
Letter of Intent for J-PARC Heavy-Ion Program (J-PARC-HI) J-PARC-HI Collaboration Spokesperson H. Sako∗1,2, J.K. Ahn3, O. Busch4, T. Chujo4, P. Cirkovic5, T. Cs¨org}o6,7, G. David8, D. Devetak5, M. Djordjevic5, S. Esumi4, P. Garg9, T. Gunji10, T. Hachiya11, H. Hamagaki12, S. Hasegawa1,2, B. Hong3, S.H. Hwang13, Y. Ichikawa1, K. Imai1,2, M. Inaba14, M. Kaneta15, B.C Kim4, E.-J. Kim16, X. Luo17, H. Masui4, Y. Miake4, J. Milosevic5, D. Mishra18, L. Nadjdjerdj5, S. Nagamiya1,11,19, T. Nakamura19, M. Naruki20, K. Nishio1, T. Nonaka4, K. Oyama12, K. Ozawa21, T.R. Saito22,23, A. Sakaguchi24, T. Sakaguchi8, K. Sato4, S. Sato1,2, S. Sawada21, K. Shigaki25, S. Shimansky26, M. Shimomura27, M. Stojanovic5, H. Sugimura1, H. Tamura15, K.H. Tanaka21, Y. Tanaka12, K. Tanida1, N. Xu17,28, S. Yokkaichi11, I.-K. Yoo29, M. Kitazawa21,24, H. Fujii30, K. Fukushima31, M. Harada32, T. Hatsuda11, T. Hirano33, K. Itakura21, T. Maruyama1, K. Morita34, K. Murase31, Y. Nara35, C. Nonaka32, A. Ohnishi34, M. Oka1,36, H. Harada2, H. Hochi2, J. Kamiya2, S. Kato2, M. Kinsho2, A. Kovalenko26, Y. Liu21, K. Okabe2, M. Okamura8, P.K. Saha2, Y. Shobuda2, F. Tamura2, J. Tamura2, N. Tani2, Y. Watababe2, M. Yamamoto2, M. Yoshii21, and M. Yoshimoto2 1Advanced Science Research Center (ASRC), Japan Atomic Energy Agency (JAEA), Tokai, Japan 2J-PARC Center, Japan Atomic Energy Agency (JAEA), Tokai, Japan 3Department of Physics, Korea University, Seoul, Korea 4Center for Integrated Research in Fundamental Science and 1 Engineering (CiRfSE), University of Tsukuba, Tsukuba, Japan 5Faculty -
Multiparticle Correlations in Pb--Pb Collisions at \Snn=2.76 Tev Jocelyn Mlynarz Wayne State University
Wayne State University Wayne State University Dissertations 1-1-2014 Multiparticle Correlations In Pb--Pb Collisions At \snn=2.76 Tev Jocelyn Mlynarz Wayne State University, Follow this and additional works at: http://digitalcommons.wayne.edu/oa_dissertations Part of the Nuclear Commons Recommended Citation Mlynarz, Jocelyn, "Multiparticle Correlations In Pb--Pb Collisions At \snn=2.76 Tev" (2014). Wayne State University Dissertations. Paper 904. This Open Access Dissertation is brought to you for free and open access by DigitalCommons@WayneState. It has been accepted for inclusion in Wayne State University Dissertations by an authorized administrator of DigitalCommons@WayneState. MULTIPARTICLE CORRELATIONS IN p PB{PB COLLISIONS AT sNN =2.76 TEV by JOCELYN MLYNARZ DISSERTATION Submitted to the Graduate School of Wayne State University, Detroit, Michigan in partial fulfillment of the requirements for the degree of DOCTOR OF PHILOSOPHY 2014 MAJOR: PHYSICS Approved by: Advisor Date DEDICATION To my dear wife, Karen Stover, for giving me the courage and strength to see this to the end. ii ACKNOWLEDGMENTS A doctorate is an endeavor that cannot be undertaken in a vacuum, and consequently a great number of people deserve my gratitude for teaching me, advising me and showing me the principles and workings of research in physics in general and relativistic heavy ions in particular. Foremost among these is my advisor, Dr. Sergei Voloshin, who granted me with the knowledge and guidance I needed to advance my research. He gave me the chance to reach this point of my academic career, and I can only hope that I will live up to the full potential of the opportunities that he provided me.