Colsell, Adam Master's Thesis.Pdf (4.393Mb)
Total Page:16
File Type:pdf, Size:1020Kb
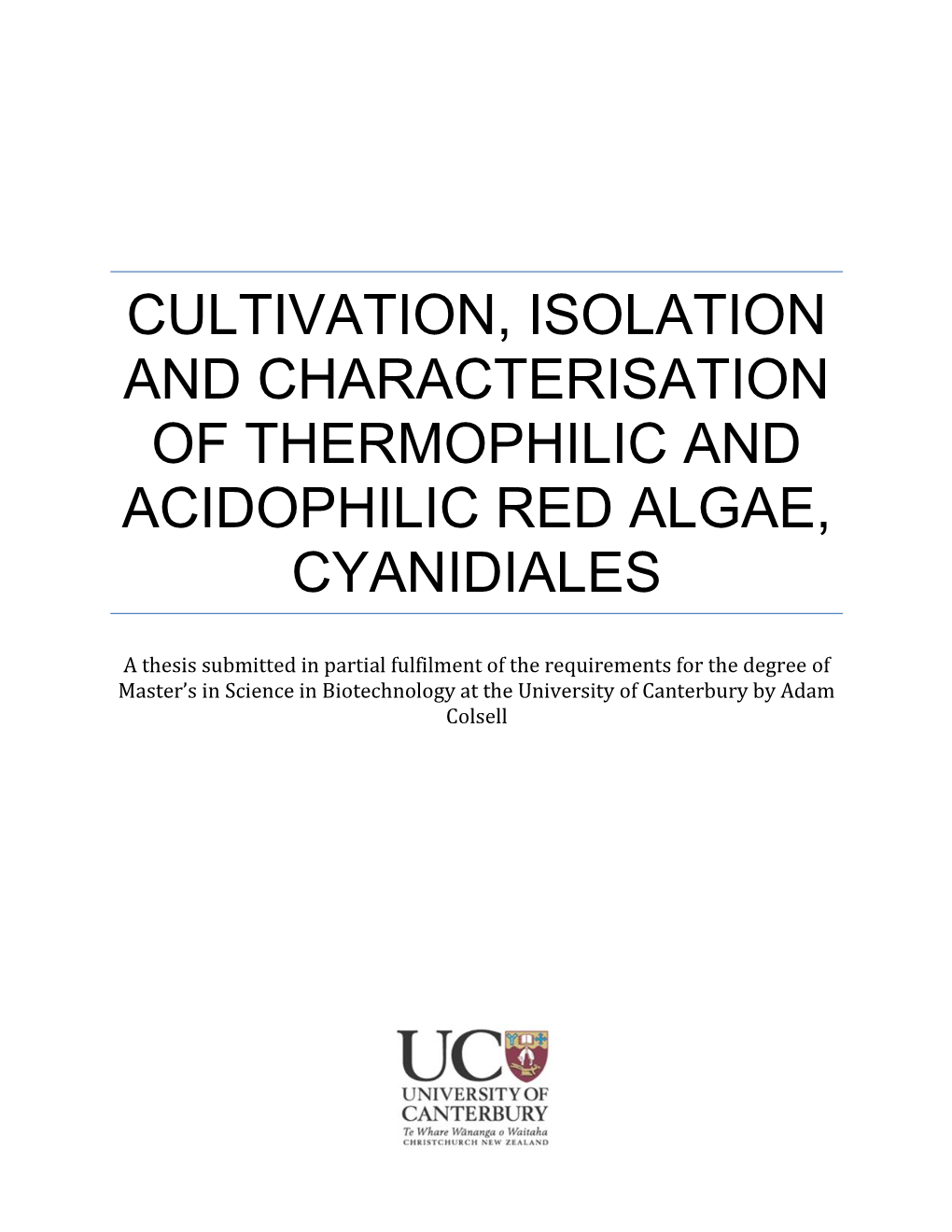
Load more
Recommended publications
-
ANALYSIS of Rbcl SEQUENCES REVEALS the GLOBAL BIODIVERSITY, COMMUNITY STRUCTURE, and BIOGEOGRAPHICAL PATTERN of THERMOACIDOPHILIC RED ALGAE (CYANIDIALES)1
J. Phycol. 51, 682–694 (2015) © 2015 Phycological Society of America DOI: 10.1111/jpy.12310 ANALYSIS OF rbcL SEQUENCES REVEALS THE GLOBAL BIODIVERSITY, COMMUNITY STRUCTURE, AND BIOGEOGRAPHICAL PATTERN OF THERMOACIDOPHILIC RED ALGAE (CYANIDIALES)1 Chia-Jung Hsieh Department of Life Science, Tunghai University, Taichung 40704, Taiwan Shing Hei Zhan Department of Zoology, University of British Columbia, Vancouver, BC, Canada V6T 1Z4 Yiching Lin Department of Life Science, Tunghai University, Taichung 40704, Taiwan Sen-Lin Tang Biodiversity Research Center, Academia Sinica, Taipei 11529, Taiwan and Shao-Lun Liu2 Department of Life Science, Tunghai University, Taichung 40704, Taiwan Thermoacidophilic cyanidia (Cyanidiales) are the the first examination of the global species primary photosynthetic eukaryotes in volcanic areas. diversity and biogeographic affinity of cyanidia. These red algae also serve as important model Additionally, our data illuminate the influence of organisms for studying life in extreme habitats. microhabitat type on Cyanidiales diversity and The global biodiversity and community structure highlight intriguing questions for future ecological of Cyanidiales remain unclear despite previous research. sampling efforts. Here, we surveyed the Cyanidiales Key index words: biodiversity; biogeography; commu- biodiversity in the Tatun Volcano Group (TVG) nity; cyanidia; Cyanidiales; microhabitats; rbcL area in Taiwan using environmental DNA sequencing. We generated 174 rbcL sequences from Abbreviations: ABGD, automated barcode gap -
Diversity and Evolution of Algae: Primary Endosymbiosis
CHAPTER TWO Diversity and Evolution of Algae: Primary Endosymbiosis Olivier De Clerck1, Kenny A. Bogaert, Frederik Leliaert Phycology Research Group, Biology Department, Ghent University, Krijgslaan 281 S8, 9000 Ghent, Belgium 1Corresponding author: E-mail: [email protected] Contents 1. Introduction 56 1.1. Early Evolution of Oxygenic Photosynthesis 56 1.2. Origin of Plastids: Primary Endosymbiosis 58 2. Red Algae 61 2.1. Red Algae Defined 61 2.2. Cyanidiophytes 63 2.3. Of Nori and Red Seaweed 64 3. Green Plants (Viridiplantae) 66 3.1. Green Plants Defined 66 3.2. Evolutionary History of Green Plants 67 3.3. Chlorophyta 68 3.4. Streptophyta and the Origin of Land Plants 72 4. Glaucophytes 74 5. Archaeplastida Genome Studies 75 Acknowledgements 76 References 76 Abstract Oxygenic photosynthesis, the chemical process whereby light energy powers the conversion of carbon dioxide into organic compounds and oxygen is released as a waste product, evolved in the anoxygenic ancestors of Cyanobacteria. Although there is still uncertainty about when precisely and how this came about, the gradual oxygenation of the Proterozoic oceans and atmosphere opened the path for aerobic organisms and ultimately eukaryotic cells to evolve. There is a general consensus that photosynthesis was acquired by eukaryotes through endosymbiosis, resulting in the enslavement of a cyanobacterium to become a plastid. Here, we give an update of the current understanding of the primary endosymbiotic event that gave rise to the Archaeplastida. In addition, we provide an overview of the diversity in the Rhodophyta, Glaucophyta and the Viridiplantae (excluding the Embryophyta) and highlight how genomic data are enabling us to understand the relationships and characteristics of algae emerging from this primary endosymbiotic event. -
Biodata of Hwan Su Yoon, Author (With Co-Author Giuseppe C
Biodata of Hwan Su Yoon, author (with co-author Giuseppe C. Zuccarello and Debashish Bhattacharya) of “Evolutionary History and Taxonomy of Red Algae” Dr. Hwan Su Yoon is currently a Senior Research Scientist at the Bigelow Laboratory for Ocean Sciences. He obtained his Ph.D. from Chungnam National University, Korea, in 1999 under the supervision of Prof. Sung Min Boo, and thereafter joined the lab of Debashish Bhattacharya at the University of Iowa. His research interests are in the areas of plastid evolution of chromalveolates, genome evolution of Paulinella, and taxonomy and phylogeny of red algae. E-mail: [email protected] Dr. Giuseppe C. Zuccarello is currently a Senior Lecturer at Victoria University of Wellington. He obtained his Ph.D. from the University of California, Berkeley, in 1993 under the supervision of Prof. John West. His research interests are in the area of algal evolution and speciation. E-mail: [email protected] Hwan Su Yoon Giuseppe C. Zuccarello 25 J. Seckbach and D.J. Chapman (eds.), Red Algae in the Genomic Age, Cellular Origin, Life in Extreme Habitats and Astrobiology 13, 25–42 DOI 10.1007/978-90-481-3795-4_2, © Springer Science+Business Media B.V. 2010 26 Hwan SU YOON ET AL. Dr. Debashish Bhattacharya is currently a Professor at Rutgers University in the Department of Ecology, Evolution and Natural Resources. He obtained his Ph.D. from Simon Fraser University, Burnaby, Canada, in 1989 under the supervision of Prof. Louis Druehl. The Bhattacharya lab has broad interests in algal evolution, endosymbiosis, comparative and functional genomics, and microbial diversity. -
Prevalent Ph Controls the Capacity of Galdieria Maxima to Use Ammonia and Nitrate As a Nitrogen Source
This is a repository copy of Prevalent pH Controls the Capacity of Galdieria maxima to Use Ammonia and Nitrate as a Nitrogen Source. White Rose Research Online URL for this paper: https://eprints.whiterose.ac.uk/156220/ Version: Accepted Version Article: Davis, Seth Jon orcid.org/0000-0001-5928-9046, Iovinella, Manuela, Carbone, Dora Allegra et al. (4 more authors) (2020) Prevalent pH Controls the Capacity of Galdieria maxima to Use Ammonia and Nitrate as a Nitrogen Source. Plant Physiology and Metabolism. Reuse Items deposited in White Rose Research Online are protected by copyright, with all rights reserved unless indicated otherwise. They may be downloaded and/or printed for private study, or other acts as permitted by national copyright laws. The publisher or other rights holders may allow further reproduction and re-use of the full text version. This is indicated by the licence information on the White Rose Research Online record for the item. Takedown If you consider content in White Rose Research Online to be in breach of UK law, please notify us by emailing [email protected] including the URL of the record and the reason for the withdrawal request. [email protected] https://eprints.whiterose.ac.uk/ ARTICLE PREVALENT PH CONTROLS THE CAPACITY OF GALDIERIA MAXIMA TO USE AMMONIA AND NITRATE AS A NITROGEN SOURCE. IOVINELLA M.1, CARBONE DA.2, CIOPPA D. 2, DAVIS S.J.1, INNANGI M. 3, ESPOSITO S. 3, CINIGLIA C.3 1 Department of BioloGy, University of York, YO105DD York UK 2Department of BioloGy, University of Naples Federico II, 80126 Naples Italy 3Department of Environmental, BioloGical and Pharmaceutical Science and TechnoloGy, University of Campania “L. -
Diversity and Evolution of Algae: Primary Endosymbiosis
Author's personal copy CHAPTER TWO Diversity and Evolution of Algae: Primary Endosymbiosis Olivier De Clerck1, Kenny A. Bogaert, Frederik Leliaert Phycology Research Group, Biology Department, Ghent University, Krijgslaan 281 S8, 9000 Ghent, Belgium 1Corresponding author: E-mail: [email protected] Contents 1. Introduction 56 1.1. Early Evolution of Oxygenic Photosynthesis 56 1.2. Origin of Plastids: Primary Endosymbiosis 58 2. Red Algae 61 2.1. Red Algae Defined 61 2.2. Cyanidiophytes 63 2.3. Of Nori and Red Seaweed 64 3. Green Plants (Viridiplantae) 66 3.1. Green Plants Defined 66 3.2. Evolutionary History of Green Plants 67 3.3. Chlorophyta 68 3.4. Streptophyta and the Origin of Land Plants 72 4. Glaucophytes 74 5. Archaeplastida Genome Studies 75 Acknowledgements 76 References 76 Abstract Oxygenic photosynthesis, the chemical process whereby light energy powers the conversion of carbon dioxide into organic compounds and oxygen is released as a waste product, evolved in the anoxygenic ancestors of Cyanobacteria. Although there is still uncertainty about when precisely and how this came about, the gradual oxygenation of the Proterozoic oceans and atmosphere opened the path for aerobic organisms and ultimately eukaryotic cells to evolve. There is a general consensus that photosynthesis was acquired by eukaryotes through endosymbiosis, resulting in the enslavement of a cyanobacterium to become a plastid. Here, we give an update of the current understanding of the primary endosymbiotic event that gave rise to the Archaeplastida. In addition, we provide an overview of the diversity in the Rhodophyta, Glaucophyta and the Viridiplantae (excluding the Embryophyta) and highlight how genomic data are enabling us to understand the relationships and characteristics of algae emerging from this primary endosymbiotic event. -
Comparison of Galdieria Growth and Photosynthetic
Carbone et al. AMB Expr (2020) 10:170 https://doi.org/10.1186/s13568-020-01110-7 ORIGINAL ARTICLE Open Access Comparison of Galdieria growth and photosynthetic activity in diferent culture systems Dora Allegra Carbone1*, Giuseppe Olivieri2,3, Antonino Pollio4 and Michael Melkonian5,6 Abstract In the last years, the acidothermophilic red microalga Galdieria sulphuraria has been increasingly studied for industrial applications such as wastewater treatment, recovery of rare earth elements, production of phycobilins. However, even now it is not possible an industrial cultivation of this organism because biotechnological research on G. sulphuraria and allied species is relatively recent and fragmented. Having in mind a possible scale-up for commercial applications, we have compared the growth and photosynthetic performance of G. sulphuraria in four suspended systems (Inclined bubble column, Decanter Laboratory Flask, Tubular Bioreactor, Ultra-fat plate bioreactor) and one immobilized system (Twin Layer Sytem). The results showed that G. sulphuraria had the highest growth, productivity and photosynthetic performance, when grown on the immobilized system, which also ofers some economics advantages. Keywords: Galdieria sulphuraria, photobioreactors, biomass, comparison Keypoints 2007; Toplin et al.2008; Castenholz and Mcdermott 2010; Ciniglia et al. 2014; Ciniglia et al. 2017) Comparison of diferent microalgal cultivation sys- Tese extreme environmental conditions strongly limit tems (suspended and immobilized) contaminations that are prevalent in open microalgal Analysis of growth and photosynthetic performance mass cultivation systems. In consequence, these organ- Immobilized cultivation on the Twin layer system isms are of considerable interest for commercial applica- showed the best performance with respect to growth tions (Carfagna et al. 2018; Carbone et al. -
Growth Under Different Trophic Regimes and Synchronization of the Red Microalga Galdieria Sulphuraria
biomolecules Article Growth under Different Trophic Regimes and Synchronization of the Red Microalga Galdieria sulphuraria Vít Náhlík 1,2,†, Vilém Zachleder 1,†,Mária Cˇ ížková 1 , KateˇrinaBišová 1 , Anjali Singh 1 , Dana Mezricky 3, Tomáš Rezankaˇ 4 and Milada Vítová 1,* 1 Centre Algatech, Laboratory of Cell Cycles of Algae, Institute of Microbiology of the Czech Academy of Sciences, Novohradská 237, 379 81 Tˇreboˇn,Czech Republic; [email protected] (V.N.); [email protected] (V.Z.); [email protected] (M.C.);ˇ [email protected] (K.B.); [email protected] (A.S.) 2 Faculty of Science, University of South Bohemia, Branišovská 1760, 370 05 Ceskˇ é Budˇejovice,Czech Republic 3 Department of Medical and Pharmaceutical Biotechnology, IMC University of Applied Sciences, Piaristengasse 1, 3500 Krems, Austria; [email protected] 4 Institute of Microbiology of the Czech Academy of Sciences, Vídeˇnská 1083, 142 20 Prague, Czech Republic; [email protected] * Correspondence: [email protected] † These two authors contributed to the study equally. Abstract: The extremophilic unicellular red microalga Galdieria sulphuraria (Cyanidiophyceae) is able to grow autotrophically, or mixo- and heterotrophically with 1% glycerol as a carbon source. The alga divides by multiple fission into more than two cells within one cell cycle. The optimal conditions of − − ◦ light, temperature and pH (500 µmol photons m 2 s 1, 40 C, and pH 3; respectively) for the strain Galdieria sulphuraria (Galdieri) Merola 002 were determined as a basis for synchronization experiments. Citation: Náhlík, V.; Zachleder, V.; For synchronization, the specific light/dark cycle, 16/8 h was identified as the precondition for Cížková,ˇ M.; Bišová, K.; Singh, A.; investigating the cell cycle. -
Using Minion Nanopore Sequencing to Generate a De Novo Eukaryotic Draft Genome
bioRxiv preprint doi: https://doi.org/10.1101/076208; this version posted September 20, 2016. The copyright holder for this preprint (which was not certified by peer review) is the author/funder, who has granted bioRxiv a license to display the preprint in perpetuity. It is made available under aCC-BY-NC-ND 4.0 International license. Using MinION nanopore sequencing to generate a de novo eukaryotic draft genome: preliminary physiological and genomic description of the extremophilic red alga Galdieria sulphuraria strain SAG 107.79 Amanda M. Davis, Manuela Iovinella, Sally James, Thomas Robshaw, Jennifer R. Dodson, Lorenzo Herrero-Davila, James H. Clark, Maria Agapiou, Simon J. McQueen-Mason, Gabriele Pinto, Claudia Ciniglia, James P. J. Chong, Peter D. Ashton, Seth J. Davis Abstract We report here the de novo assembly of a eukaryotic genome using only MinION nanopore DNA sequence data by examining a novel Galdieria sulphuraria genome: strain SAG 107.79. This extremophilic red alga was targeted for full genome sequencing as we found that it could grow on a wide variety of carbon sources and could uptake several precious and rare-earth metals, which places it as an interesting biological target for disparate industrial biotechnological uses. Phylogenetic analysis clearly places this as a species of G. sulphuraria. Here we additionally show that the genome assembly generated via nanopore long read data was of a high quality with regards to low total number of contiguous DNA sequences and long length of assemblies. Collectively, the MinION platform looks to rival other competing approaches for de novo genome acquisition with available informatics tools for assembly. -
The Thermo- Acidophilic Cyanidiophyceae (Cyanidiales)”
Biodata of Valérie Reeb and Debashish Bhattacharya, authors of “The Thermo- acidophilic Cyanidiophyceae (Cyanidiales)” Dr. Valérie Reeb is currently a Postdoctoral Research Associate at the Department of Biological Science in the University of Iowa, USA. She obtained her Ph.D. from Duke University in 2005 and continued her postdoctoral studies at Duke University and the University of Iowa. Dr. Reeb’s scientific interests are in the areas of evolution of algae, protists and fungi, and molecular phylogeny of Cyanidiales (red Algae) and lichen forming-fungi. E-mail: valerie-reeb @ uiowa.edu Dr. Debashish Bhattacharya is currently a Professor in the Department of Ecology, Evolution and Natural Resources at Rutgers University. He obtained his Ph.D. from Simon Fraser University, Burnaby, Canada under the supervision of Prof. Louis Druehl. The Bhattacharya lab has broad interests in algal evolution, endosymbiosis, comparative and functional genomics, and microbial diversity. E-mail: [email protected] Valérie Reeb Debashish Bhattacharya 409 J. Seckbach and D.J. Chapman (eds.), Red Algae in the Genomic Age, Cellular Origin, Life in Extreme Habitats and Astrobiology 13, 409–426 DOI 10.1007/978-90-481-3795-4_22, © Springer Science+Business Media B.V. 2010 THE THERMO-ACIDOPHILIC CYANIDIOPHYCEAE (CYANIDIALES) VALÉRIE REEB1 AND Debashish Bhattacharya2 1Department of Biological Sciences and the Roy J. Carver Center for Comparative Genomics, University of Iowa, Iowa City, Iowa 52242, USA 2Department of Ecology, Evolution and Natural Resources, Rutgers University, NJ 08901, USA 1. Introduction 1.1. BACKGROUND Extremophiles are organisms that thrive in environments previously thought inhospitable to life because the physicochemical characteristics fall outside the range tolerated by human cells. -
Davis2, Diana Cioppa1 and Claudia Ciniglia4*
This is a repository copy of A Spotlight on Rad52 in Cyanidiophytina (Rhodophyta) : A Relic in Algal Heritage. White Rose Research Online URL for this paper: http://eprints.whiterose.ac.uk/142606/ Version: Accepted Version Article: Del Mondo, Angelo, Iovinella, Manuela, Petriccione, Milena et al. (4 more authors) (2019) A Spotlight on Rad52 in Cyanidiophytina (Rhodophyta) : A Relic in Algal Heritage. Plants. 46. https://doi.org/10.3390/plants8020046 Reuse This article is distributed under the terms of the Creative Commons Attribution (CC BY) licence. This licence allows you to distribute, remix, tweak, and build upon the work, even commercially, as long as you credit the authors for the original work. More information and the full terms of the licence here: https://creativecommons.org/licenses/ Takedown If you consider content in White Rose Research Online to be in breach of UK law, please notify us by emailing [email protected] including the URL of the record and the reason for the withdrawal request. [email protected] https://eprints.whiterose.ac.uk/ 1 A SPOTLIGHT ON RAD52 IN CYANIDIOPHYTINA (RHODOPHYTA): A 2 RELIC IN ALGAL HERITAGE 3 Angelo Del Mondo1, Manuela Iovinella2, Milena Petriccione3, Angelina Nunziata3, 4 Seth J. Davis2, Diana Cioppa1 and Claudia Ciniglia4* 5 6 1Department of Biology, University of Naples Federico II, Via Cinthia 21, 80126 7 Naples, Italy. 8 2 Department of Biology, University of York, YO105DD York UK 9 3 C.R.E.A.– Council for Agricultural Research and Economics – Research Centre for 10 Olive, Citrus and Tree Fruit (OFA); Via Torrino 2; 81100 Caserta, Italy 11 4Department of Environmental, Biological and Pharmaceutical Science and 12 Technology, University of Campania “L. -
Defining the Major Lineages of Red Algae (Rhodophyta)1
J. Phycol. 42, 482–492 (2006) r 2006 Phycological Society of America DOI: 10.1111/j.1529-8817.2006.00210.x DEFINING THE MAJOR LINEAGES OF RED ALGAE (RHODOPHYTA)1 Hwan Su Yoon Department of Biological Sciences and Roy J. Carver Center for Comparative Genomics, University of Iowa, 468 Biology Building, Iowa City, Iowa 52242, USA Kirsten M. Mu¨ller Department of Biology, University of Waterloo, Waterloo, ON, Canada N2L 3G1 Robert G. Sheath Office of the Provost, California State University San Marcos, San Marcos, California 92096, USA Franklyn D. Ott 905 NE Hilltop Drive, Topeka, Kansas 66617, USA and Debashish Bhattacharya2 Department of Biological Sciences and Roy J. Carver Center for Comparative Genomics, University of Iowa, 446 Biology Building, Iowa City, Iowa 52242, USA Previous phylogenetic studies of the Rhodophyta dospora,andRufusia). We also describe a new have provided a framework for understanding red order, Rhodellales, and a new family, Rhodellaceae algal phylogeny, but there still exists the need for a (with Rhodella, Dixoniella, and Glaucosphaera). comprehensive analysis using a broad sampling of Key index words: Bangiophyceae; Compsopogono- taxa and sufficient phylogenetic information to phyceae; Cyanidiophyceae; Florideophyceae; clearly define the major lineages. In this study, we Porphyridiophycae; red algal lineages; Rhod- determined 48 sequences of the PSI P700 chl a ellophyceae; Rhodophyta; Stylonematophyceae apoprotein A1 (psaA) and rbcL coding regions and established a robust red algal phylogeny to identify Abbreviations: BPP, Bayesian posterior probabili- the major clades. The tree included most of the lin- ties; ML, maximum likelihood; MP, maximum par- eages of the Bangiophyceae (25 genera, 48 taxa). -
Potential Causes and Consequences Of
Cho et al. BMC Evolutionary Biology (2020) 20:112 https://doi.org/10.1186/s12862-020-01677-6 RESEARCH ARTICLE Open Access Potential causes and consequences of rapid mitochondrial genome evolution in thermoacidophilic Galdieria (Rhodophyta) Chung Hyun Cho1†, Seung In Park1†, Claudia Ciniglia2, Eun Chan Yang3, Louis Graf1, Debashish Bhattacharya4 and Hwan Su Yoon1* Abstract Background: The Cyanidiophyceae is an early-diverged red algal class that thrives in extreme conditions around acidic hot springs. Although this lineage has been highlighted as a model for understanding the biology of extremophilic eukaryotes, little is known about the molecular evolution of their mitochondrial genomes (mitogenomes). Results: To fill this knowledge gap, we sequenced five mitogenomes from representative clades of Cyanidiophyceae and identified two major groups, here referred to as Galdieria-type (G-type) and Cyanidium-type (C-type). G-type mitogenomes exhibit the following three features: (i) reduction in genome size and gene inventory, (ii) evolution of unique protein properties including charge, hydropathy, stability, amino acid composition, and protein size, and (iii) distinctive GC-content and skewness of nucleotides. Based on GC-skew-associated characteristics, we postulate that unidirectional DNA replication may have resulted in the rapid evolution of G-type mitogenomes. Conclusions: The high divergence of G-type mitogenomes was likely driven by natural selection in the multiple extreme environments that Galdieria species inhabit combined with their