Hafnium Isotopes in Zircons Document the Gradual Onset of Mobile- Lid Tectonics
Total Page:16
File Type:pdf, Size:1020Kb
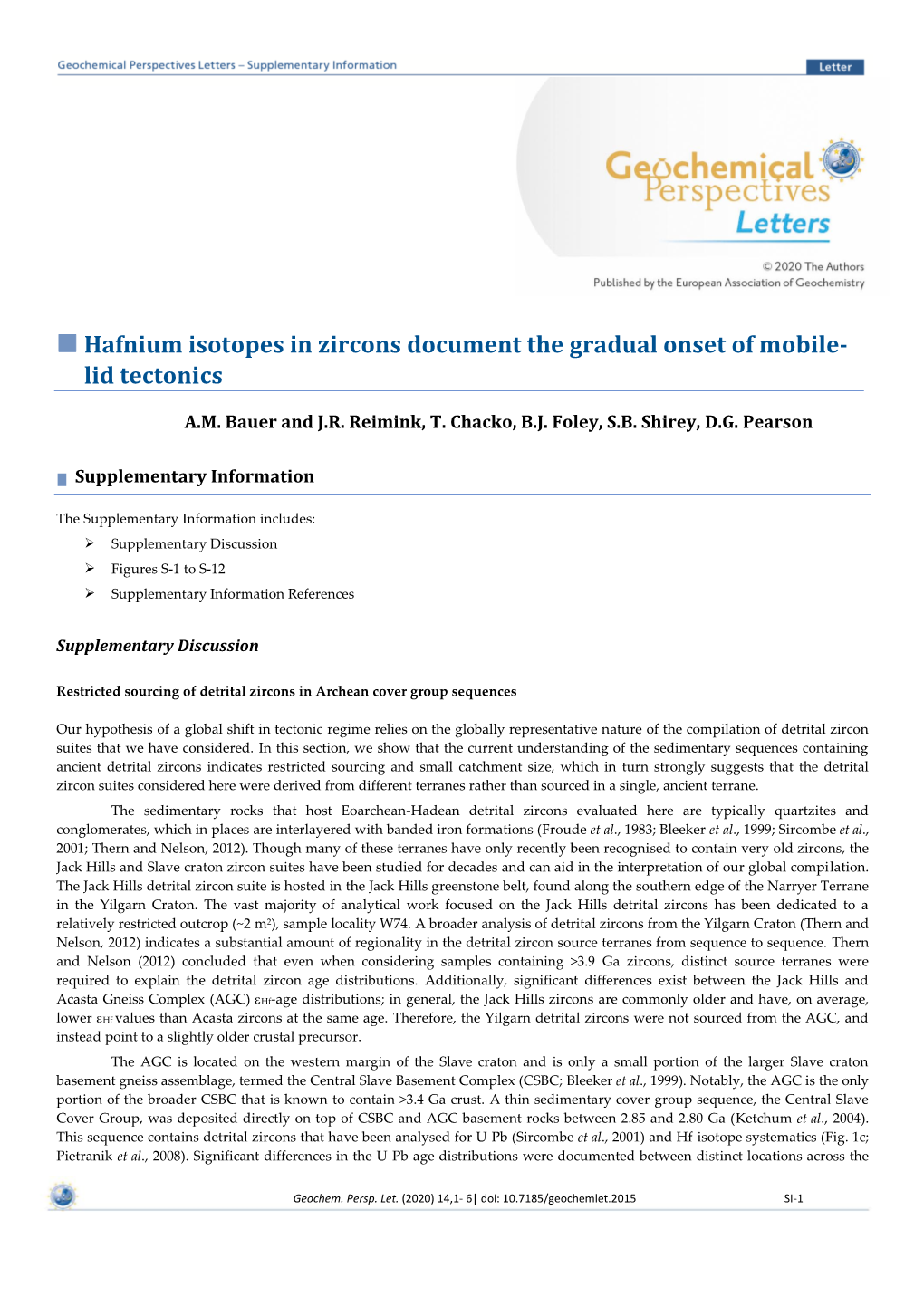
Load more
Recommended publications
-
Earth's Oldest Rocks
Chapter 12 The Oldest Terrestrial Mineral Record: Thirty Years of Research on Hadean Zircon From Jack Hills, Western Australia Aaron J. Cavosie1, John W. Valley2 and Simon A. Wilde3 1Curtin University, Perth, WA, Australia; 2University of WisconsineMadison, Madison, WI, United States; 3Curtin University of Technology, Perth, WA, Australia Chapter Outline 1. Introduction 255 3.4.1 Lithium 266 2. Jack Hills Metasedimentary Rocks 256 3.4.2 Ti Thermometry 266 2.1 Age of Deposition 257 3.4.3 Rare Earth Elements, Yttrium and Phosphorous 266 2.2 Metamorphism 258 3.4.4 Other Trace Elements (Al, Sc, Sm/Nd, Xe) 267 2.3 Geology of Adjacent Rocks 258 3.5 Hafnium Isotopic Compositions 267 3. Studies of Jack Hills Zircon 259 3.6 Mineral Inclusion Studies 268 3.1 Images of Jack Hills Zircon 259 4. Early Earth Processes Recorded by Jack Hills Zircon 270 3.2 Age of the Hadean Zircon Population 259 4.1 Derivation of Jack Hills Zircon From Early Mafic 3.2.1 The UePb Story 259 Crust (εHf) 270 3.2.2 U Abundance, Radiation Damage, and Pb Loss 261 4.2 Existence of >4300 Ma Granitoid 270 3.2.3 The Oldest Grains in the Jack Hills 262 4.3 Significance of Surface Alteration on the Early Earth 271 3.2.4 Distribution of Hadean Grains 264 4.4 Impact Events Recorded in Jack Hills Zircon? 271 3.3 Oxygen Isotopes 264 Acknowledgments 273 3.4 Trace Elements 266 References 273 1. INTRODUCTION Little is known of the early Earth because of the absence of a rock record for the first 500 million years after accretion. -
Proterozoic Deformation in the Northwest of the Archean Yilgarn Craton, Western Australia Catherine V
Available online at www.sciencedirect.com Precambrian Research 162 (2008) 354–384 Proterozoic deformation in the northwest of the Archean Yilgarn Craton, Western Australia Catherine V. Spaggiari a,∗, Jo-Anne Wartho b,1, Simon A. Wilde b a Geological Survey of Western Australia, Department of Industry and Resources, 100 Plain Street, East Perth, Western Australia 6004, Australia b Department of Applied Geology, Curtin University, GPO Box U1987, Perth, Western Australia 6845, Australia Received 31 January 2007; received in revised form 19 September 2007; accepted 16 October 2007 Abstract The Narryer Terrane within the northwestern Yilgarn Craton contains the oldest crust in Australia. The Jack Hills greenstone belt is located within the southern part of the Narryer Terrane, and structures cutting it and surrounding rocks have been dated using the 40Ar/39Ar technique. The results show that east-trending, dextral, transpressive shearing was related to the 1830–1780 Ma Capricorn Orogeny, followed by further deformation and/or cooling between c. 1760 and 1740 Ma. These results confirm that major deformation has affected the northwestern part of the Yilgarn Craton in an intracratonic setting during the Proterozoic. Proterozoic structures have been interpreted to extend south beyond the Narryer Terrane into the northern part of the Youanmi Terrane (Murchison Domain), and include the Yalgar Fault, previously interpreted as the boundary between the Narryer and Youanmi Terranes. Terrane amalgamation pre-dated the emplacement of c. 2660 Ma granites in both terranes, and the current expression of the Yalgar Fault must represent a younger, reworked, post-amalgamation structure, possibly controlled by the tectonic boundary. However, new aeromagnetic and gravity imagery does not show the eastern part of the Yalgar Fault as a major structure. -
Heterogeneous Hadean Crust with Ambient Mantle Affinity Recorded in Detrital Zircons of the Green Sandstone Bed, South Africa
Heterogeneous Hadean crust with ambient mantle affinity recorded in detrital zircons of the Green Sandstone Bed, South Africa Nadja Drabona,1,2, Benjamin L. Byerlyb,3, Gary R. Byerlyc, Joseph L. Woodend,4, C. Brenhin Kellere, and Donald R. Lowea aDepartment of Geological Sciences, Stanford University, Stanford, CA 94305; bDepartment of Earth Sciences, University of California, Santa Barbara, CA 93106; cDepartment of Geology and Geophysics, Louisiana State University, Baton Rouge, LA 70803; dPrivate address, Marietta, GA 30064; and eDepartment of Earth Sciences, Dartmouth College, Hanover, NH 03755 Edited by Albrecht W. Hofmann, Max Planck Institute for Chemistry, Mainz, Germany, and approved January 4, 2021 (received for review March 10, 2020) The nature of Earth’s earliest crust and the processes by which it While the crustal rocks in which Hadean zircon formed have formed remain major issues in Precambrian geology. Due to the been lost, the trace and rare earth element (REE) geochemistry of absence of a rock record older than ∼4.02 Ga, the only direct re- these zircons can be used to characterize their parental magma cord of the Hadean is from rare detrital zircon and that largely compositions. Zircon crystallizes as a ubiquitous accessory mineral from a single area: the Jack Hills and Mount Narryer region of in silica-rich, differentiated magmas formed in a number of crustal Western Australia. Here, we report on the geochemistry of environments. Since zircon compositions are influenced by varia- Hadean detrital zircons as old as 4.15 Ga from the newly discov- tions in melt composition, coexisting mineral assemblage, and trace ered Green Sandstone Bed in the Barberton greenstone belt, element partitioning as a function of magmatic processes, temper- South Africa. -
Potentially Biogenic Carbon Preserved in a 4.1 Billion-Year-Old Zircon
Potentially biogenic carbon preserved in a 4.1 billion-year-old zircon Elizabeth A. Bella,1, Patrick Boehnkea, T. Mark Harrisona,1, and Wendy L. Maob aDepartment of Earth, Planetary, and Space Sciences, University of California, Los Angeles, CA 90095; and bSchool of Earth, Energy, and Environmental Sciences, Stanford University, Stanford, CA 94305 Contributed by T. Mark Harrison, September 4, 2015 (sent for review July 31, 2015) Evidence of life on Earth is manifestly preserved in the rock record. Results However, the microfossil record only extends to ∼3.5 billion years From an initial population of over 10,000 Jack Hills zircons (6), (Ga), the chemofossil record arguably to ∼3.8 Ga, and the rock we examined 656 grains with ages over 3.8 Ga for the presence of record to 4.0 Ga. Detrital zircons from Jack Hills, Western Australia graphitic inclusions. The zircons were mounted in epoxy and range in age up to nearly 4.4 Ga. From a population of over 10,000 polished to expose their interiors. The search protocol included > Jack Hills zircons, we identified one 3.8-Ga zircon that contains an initial screening for opaque inclusions using transmitted light primary graphite inclusions. Here, we report carbon isotopic mea- microscopy. Seventy-nine candidates thus identified were then ± surements on these inclusions in a concordant, 4.10 0.01-Ga targeted for Raman spectroscopy from which we documented zircon. We interpret these inclusions as primary due to their enclo- two zircons containing partially disordered graphite (Fig. 1, In- sure in a crack-free host as shown by transmission X-ray microscopy δ13 − ± ‰ set) beneath their polished surfaces (RSES 81-10.14 in a cracked and their crystal habit. -
1 Geochronology of Hadean Zircon Grains from the Jack Hills, Western Australia 1 Constrained by Quantitative Scanning Ion Imagin
*Revised manuscript with no changes marked Click here to view linked References 1 Geochronology of Hadean zircon grains from the Jack Hills, Western Australia 2 constrained by quantitative scanning ion imaging 3 4 J. J. Bellucci1*, A. A. Nemchin1,2, M. J. Whitehouse1, R.B. Kielman1, J. F. Snape1, and 5 R.T. Pidgeon2 6 7 *Corresponding author, email address: [email protected] 8 9 1Department of Geosciences, Swedish Museum of Natural History, SE-104 05 10 Stockholm, Sweden 11 2 Department of Applied Geology, Curtin University, Perth, WA 6845, Australia 12 13 Abstract 14 Five Hadean (>4 Ga) aged zircon grains from the Jack Hills metasedimentary belt 15 have been investigated by a secondary ion mass spectrometry scanning ion image 16 technique. This technique has the ability to obtain accurate and precise full U-Pb 17 systematics on a scale <5 mm, as well as document the spatial distribution of U, Th and 18 Pb. All five of the grains investigated here have complex cathodoluminescence patterns 19 that correlate to different U, Th, and Pb concentration domains. The age determinations 20 for these different chemical zones indicate multiple reworking events that are preserved 21 in each grain and have affected the primary crystalized zircon on the scale of <10 mm, 22 smaller than conventional ion microprobe spot analyses. In comparison to the spot 23 analyses performed on these grains, these new scanning ion images and age 24 determinations indicate that almost half of the spot analyses have intersected several age 25 and chemical domains in both fractured and unfractured parts of the individual crystals. -
Jack Hills Iron Ore Project, Murchison Region, Western Australia
AustralianGovernment Ilepar*neat ofthe Enviro:rmentand tleritage Ms Kate George Martinick BoschSeI Pty Ltd ln]trGtrE\7tri[]] Cook strcet ill z z srpzoos l!.J !!EST PERTH WA 6005 -t /q /{ Dear Ms George Murchison Metals Limited/Mining/Mu.hison region/WA.{a& Hills Iron Ore Mine and crushing and sceening plant and transport to Geraldton Port - EPBC 2O051227a Thank you for the above referral, received on 22 August 2005,for decision whether or not approval is needed under Chapter 4 of the Ea"ironment Prctectionand Biodioersity ConserustionAct 1999 GPBC AcO. fhe referal has now been considered undei the EPBC Act and I have decided that the action is not a controlled action. Approval is therefore not needed under Part 9 of the Act before +lla r-ti^n .^n nr roo.l Pleasenote that this decision only relates to the potential for significant impact on the specific matte$ of national environmental signficance protected by the Australan Covemnent under the EPBC Act. There mav be a need for separate State or Local Covemment envhonmental assessmentand approval to address potential impacts on State, regional or local environrnental values. A copy of the document recording my decision is attached for your inJormation. I have written separately to Mr FranL Sibbel of Murchison Metals Limited to advise of my decision (copy attached). aecic+>n+ qo.ro+,fl| EnvironmentAssessment Branch )5 September2005 GPO Box 787Canberra ACl 260| lelephone(02) 6274 l111 F acsimlle (02) 6274 1666 Internet:www.deh.gov.au COMMONWEALTHO! AUSTRALIA ENI,GOA,\4'Ar PRoTEcTIoN4^? BIoDIt.ERsTy C'NSERVATI1NAcT 7999 DDCISIoNTHAT ACTIoN Is NoT A CoNTRoLLEDAcTIoN I, ALEX RANKIN, Assistant Secretary, Envioffirent Assessment Bmndr, Department of the Enviro{:,$rent and Heritage, a delegate of the Minister for the Environment and Heritage for the purposes of section 75 of the Enoironnmt Protectirrftahd Biodioercity ConsetuatiotlAct 1999,decide that the proposed actiorL 6et out in the Schedule, is not a controlled action. -
Bulletin of the Geological Society of Denmark, Vol. 39/3-4 Pp. 199-211
Tectonostratigraphic terranes within Archaean gneiss complexes: examples from Western Australia and southern West Greenland ALLEN P. NVTMAN Nutman, A. P.: Tectonostratigraphic terranes within Archaean gneiss complexes: examples from Western Australia and southern West Greenland. Bull. geol. Soc. Denmark, vol. 39, pp. 199-211. Copenhagen, December 20th, 1991. https://doi.org/10.37570/bgsd-1991-39-09 New field work and isotopic data show that the Godthabsfjord region of West Greenland consists of a collage of tectonostratigraphic terranes, which evolved separately prior to tectonic juxtaposition in the late Archaean. In WesternA ustralia the Narryer Gneiss Complex, which lies on the northwesternm argin of the Yilgarn Craton, is, unlike the Godthabsfjord region, very poorly exposed (less than 1 % ). In consequence it is impossible to follow geological boundaries in this complex, and instead the complex has been studied by a very extensive use of within-grain zircon U-Pb geochronology on the ion microprobe SHRIMP. The zircon geochronology suggests that the Narryer Gneiss Complex also consists of several discrete terranes of early to mid Archaean gneisses. In both the Godthabsfjord region and the Narryer Gneiss Complex, late Archaean juxtaposition of terranes was accompanied by intrusion of crustally derived granites, deformation, and amphibolite facies metamorphism. Thus some Archaean high grade gneiss complexes consist of terranes that underwent independent evolution until they were brought together at a later time. In this respect their anatomy resembles post-Archaean orogenic belts that formed as a consequence of plate tectonic processes. Allen P. Nutman, Research School of Earth Sciences, Australian National University, G.P.O. Box 4, Canberra ACT 2601, Australia.June 8th, 1990. -
A Cool Early Earth, (2002)
A cool early Earth John W. Valley* William H. Peck* Elizabeth M. King* Department of Geology and Geophysics, University of Wisconsin, Madison, Wisconsin 53706, USA Simon A. Wilde School of Applied Geology, Curtin University of Technology, GPO Box U1987, Perth, Australia ABSTRACT No known rocks have survived from the ®rst 500 m.y. of Earth history, but studies of single zircons suggest that some continental crust formed as early as 4.4 Ga, 160 m.y. after accretion of the Earth, and that surface temperatures were low enough for liquid water. Surface temperatures are inferred from high d18O values of zircons. The range of d18O values is constant throughout the Archean (4.4±2.6 Ga), suggesting uniformity of processes and conditions. The hypothesis of a cool early Earth suggests long intervals of relatively temperate surface conditions from 4.4 to 4.0 Ga that were conducive to liquid- water oceans and possibly life. Meteorite impacts during this period may have been less frequent than previously thought. Keywords: Archean, zircon, oxygen, isotopes, meteorites, impacts. INTRODUCTION ``hell-like,'' in which any crust was destroyed and Table DR-11). Figure 2 shows histograms The young Earth (4.5±3.8 Ga) was highly by meteorite impact or melting, all water was of d18O for these samples in comparison to energetic. Sources of heat, both internal and ex- vaporized, and the atmosphere was steam rich olivine and zircon from Earth's mantle. Sev- ternal, were signi®cantly greater than at any (see Pollack, 1997). eral conclusions can be made. There is no sys- time since. -
Processes on the Young Earth and the Habitats of Early Life
EA40CH21-Arndt ARI 23 March 2012 15:30 Processes on the Young Earth and the Habitats of Early Life Nicholas T. Arndt1 and Euan G. Nisbet2 1ISTerre, CNRS UMR 5275, University of Grenoble, 38400 St-Martin d’Heres,` France; email: [email protected] 2Department of Earth Sciences, Royal Holloway, University of London, Egham Hill TW20 0EX, United Kingdom; email: [email protected] Annu. Rev. Earth Planet. Sci. 2012. 40:521–49 Keywords The Annual Review of Earth and Planetary Sciences is Hadean, Archean, komatiites, photosynthesis, crust online at earth.annualreviews.org This article’s doi: Abstract 10.1146/annurev-earth-042711-105316 Conditions at the surface of the young (Hadean and early Archean) Earth Copyright c 2012 by Annual Reviews. were suitable for the emergence and evolution of life. After an initial hot All rights reserved period, surface temperatures in the late Hadean may have been clement be- by Rice University on 04/21/14. For personal use only. 0084-6597/12/0530-0521$20.00 neath an atmosphere containing greenhouse gases over an ocean-dominated planetary surface. The first crust was mafic and it internally melted repeat- edly to produce the felsic rocks that crystallized the Jack Hills zircons. This crust was destabilized during late heavy bombardment. Plate tectonics prob- ably started soon after and had produced voluminous continental crust by Annu. Rev. Earth Planet. Sci. 2012.40:521-549. Downloaded from www.annualreviews.org the mid Archean, but ocean volumes were sufficient to submerge much of this crust. In the Hadean and early Archean, hydrothermal systems around abundant komatiitic volcanism may have provided suitable sites to host the earliest living communities and for the evolution of key enzymes. -
Internal Zoning and U–Th–Pb Chemistry of Jack Hills Detrital Zircons: a Mineral Record of Early Archean to Mesoproterozoic (4348–1576 Ma) Magmatism
Precambrian Research 135 (2004) 251–279 Internal zoning and U–Th–Pb chemistry of Jack Hills detrital zircons: a mineral record of early Archean to Mesoproterozoic (4348–1576 Ma) magmatism Aaron J. Cavosiea,∗, Simon A. Wildeb, Dunyi Liuc, Paul W. Weiblend, John W. Valleya a Department of Geology and Geophysics, University of Wisconsin, 1215 W. Dayton St., Madison, WI 53706, USA b Department of Applied Geology, Curtin University of Technology, Kent Street, Bentley, WA 6102, Australia c Institute of Geology, Chinese Academy of Geological Sciences, 26 Baiwanzhuang Road, Beijing 100037, PR China d Department of Geology and Geophysics, University of Minnesota, 310 Pillsbury Drive SE, Minneapolis, MN 55455, USA Received 26 February 2004; received in revised form 6 July 2004; accepted 3 September 2004 Abstract Magmatic processes were important on the nascent Earth during the first 500 million years (Ma) after accretion, yet the causes and timing of this early magmatism are largely unconstrained, as no rocks from this period have been discovered. Rare >4000 Ma detrital zircons from Western Australia preserve the only direct geologic evidence of this early magmatism. To understand the genesis and history of these zircons, we present the results of a combined ion and electron microprobe, and SEM study of the age, Th–U chemistry, cathodoluminescence (CL) zoning patterns, and inclusions for a population of detrital zircons from Jack Hills, Western Australia, with 207Pb/206Pb ages ranging from 4348 to 1576 Ma. The majority of the zircons preserve primary growth features discernable by CL imaging, such as oscillatory and sector zoning, have Th/U ratios from 0.1 to 1.0, and several contain granitic mineral inclusions. -
Hadean Zircon Petrochronology T
Reviews in Mineralogy & Geochemistry Vol. 83 pp. 329–363, 2017 11 Copyright © Mineralogical Society of America Hadean Zircon Petrochronology T. Mark Harrison, Elizabeth A. Bell, Patrick Boehnke Department of Earth, Planetary and Space Sciences University of California, Los Angeles Los Angeles, CA 90095 USA [email protected] [email protected] [email protected] INTRODUCTION The inspiration for this volume arose in part from a shift in perception among U–Pb geochronologists that began to develop in the late 1980s. Prior to then, analytical geochronology emphasized progressively lower blank analysis of separated accessory mineral aggregates (e.g., Krogh 1982; Parrish 1987), with results generally interpreted to reflect a singular moment in time. For example, a widespread measure of confidence in intra-analytical reliability was conformity to an MSWD (a form of χ2 test; Wendt and Carl 1991) of unity. This approach implicitly assumed that geological processes act on timescales that are short with respect to analytical errors (e.g., Schoene et al. 2015). As in situ methodologies (e.g., Compston and Pidgeon 1986; Harrison et al. 1997; Griffin et al. 2000) and increasingly well-calibrated double spikes (e.g., Amelin and Davis 2006; McLean et al. 2015) emerged, geochronologists began to move away from interpreting geological processes as a series of instantaneous episodes (e.g., Rubatto 2002). At about the same time, petrologists developed techniques that permitted in situ chemical analyses to be interpreted in terms of continuously changing pressure–temperature– time histories (e.g., Spear 1988). The recognition followed that specific mineral reactions yielded products that could be directly dated or interpreted in terms of protracted petrogenetic processes. -
Early Earth University of Maryland
Michael Brown Early Earth University of Maryland Bleeker, 2003, Lithos Gerya, 2014, Gondwana Res. Questions: Was there only one style of “Precambrian geodynamics”? Or, was the geodynamic regime on a hotter early Earth different? Did a subduction/mobile-lid plate tectonics regime only begin sometime during the (late) Archean? If so, what preceded it? Acasta Gneiss complex on the Old rocks are rare! west side of the Slave craton Earth’s undisputed oldest crustal rocks are components in the Acasta Gneiss at 4.03 Ga (Images courtesy of Ian Williams, ANU) Earth’s oldest known crustal material? Zircons in the Jack Hills metasedimentary rocks. But information from detrital minerals lacks important geological context Jack Hills, Naryer terrane, Yilgarn craton Froude et al., 1983, Nature 4.374 ± 0.006 Ga Valley et al., 2014, Nature Geosci. “Water is essential for the formation of granite and granite, in turn, is essential for the formation of continents. Earth, the only inner planet with abundant water, is the only planet with granite and continents. The Moon and the other inner planets have little or no water and no granites or continents.” Campbell & Taylor, 1983, GRL Evolution of mantle temperature Thermal history calculations by Labrosse & The decline in mantle TP from the Mesoarchean Jaupart (2007, EPSL) lead to a maximum o to the present may have been as much as 250 C, temperature (ΔT ~250oC) at 3.0 Ga and but with a spread of values similar to the present cannot be extrapolated further back in time. day (~120oC). Labrosse & Jaupart argue that ΔT was ~200oC higher than the present day at the start of mantle convection after crystallization of the last magma ocean.