Ecological Determinants of Oceanic Carbon Cycling (EDOCC)
Total Page:16
File Type:pdf, Size:1020Kb
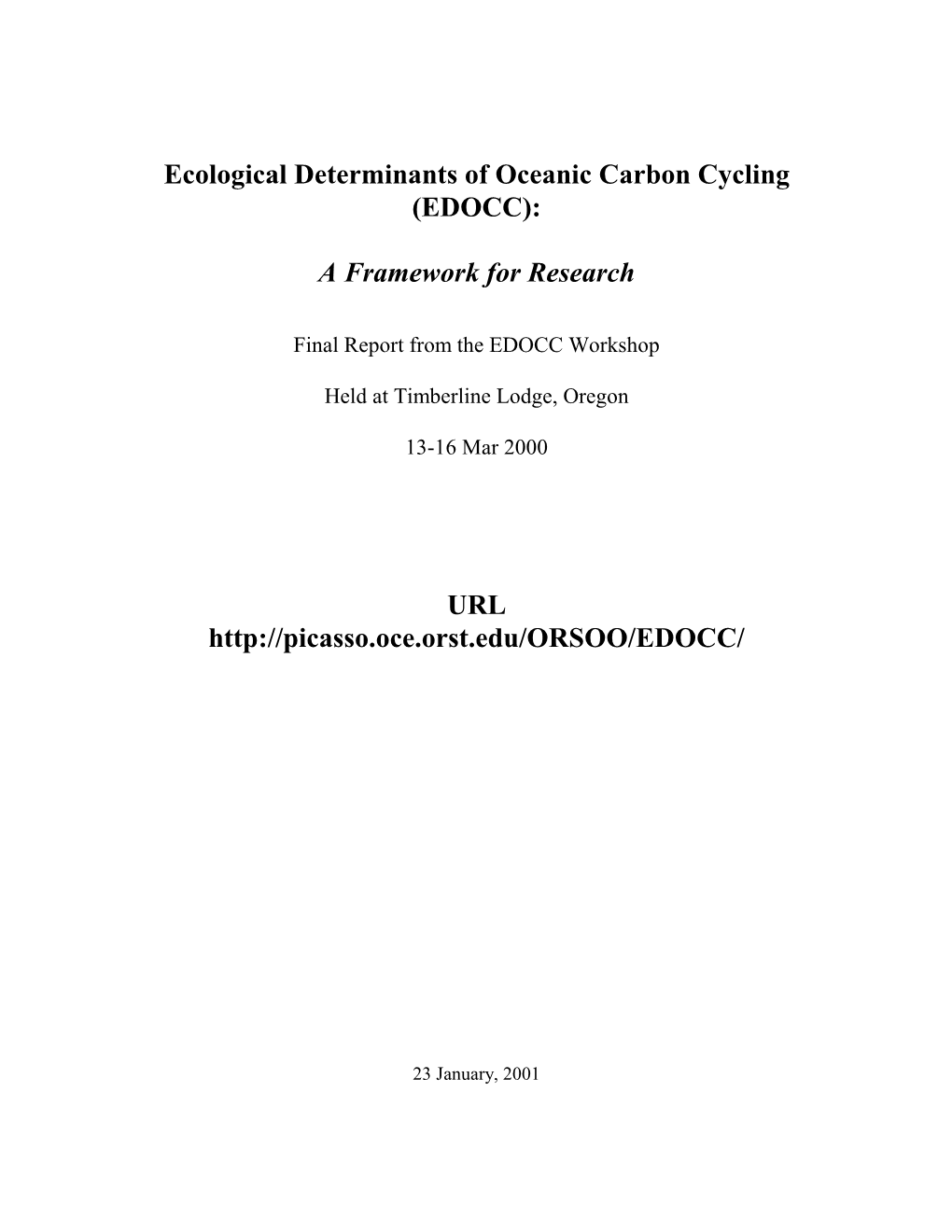
Load more
Recommended publications
-
Sodium Silicate Crops
Sodium Silicate Crops 1 Identification of Petitioned Substance 2 3 Chemical Names: CAS Numbers: 4 Sodium Silicate 1344-09-8 5 6 Other Name: Other Codes: 7 Sodium metasilicate; Sodium silicate glass; EPA PC code: 072603 (NLM, 2011a); European 8 Sodium water glass; Silicic acid, sodium salt; Inventory of Existing Commercial Chemical 9 tetrasodium orthosilicate (IPCS, 2004) Substances (EINECS) Number: 215-687-4 (IPCS, 10 2004) 11 Trade Names: 12 Waterglass, Britesil, Sikalon, Silican, Carsil, 13 Dryseq, Sodium siloconate, Star, Soluble glass, 14 Sodium polysilicate (NLM, 2011a), N® - PQ 15 Corporation (OMRI, 2011) 16 Characterization of Petitioned Substance 17 18 Composition of the Substance: 19 The basic formula of sodium silicate is Na2O· nO2Si, which represents the components of silicon dioxide (SiO2) 20 and sodium oxide (Na2O) and the varying ratios of the two in the various formulations. This ratio is commonly 21 called the molar ratio (MR), which can range from 0.5 to 4.0 for sodium silicates and varies depending on the 22 composition of the specific sodium silicate. The structural formulas of these silicates are also variable and can be 23 complex, depending on the formulation, but generally do not have distinct molecular structures (IPCS, 2004). 24 The basic structure of soluble silicates, including sodium and potassium silicates, is a trigonal planar 25 arrangement of oxygen atoms around a central silicon atom, as depicted in Figure 1 below. Physical and 26 chemical properties of sodium silicate are summarized in Table 1, on page 2. 27 28 29 30 31 32 33 34 35 Figure 1: Chemical Structure of Sodium Silicate (NLM, 2011a) 36 37 Specific Uses of the Substance: 38 39 Sodium silicate and other soluble silicates have been used in many industries since the early 19th century. -
CO2-Fixing One-Carbon Metabolism in a Cellulose-Degrading Bacterium
CO2-fixing one-carbon metabolism in a cellulose- degrading bacterium Clostridium thermocellum Wei Xionga, Paul P. Linb, Lauren Magnussona, Lisa Warnerc, James C. Liaob,d, Pin-Ching Manessa,1, and Katherine J. Choua,1 aBiosciences Center, National Renewable Energy Laboratory, Golden, CO 80401; bDepartment of Chemical and Biomolecular Engineering, University of California, Los Angeles, CA 90095; cNational Bioenergy Center, National Renewable Energy Laboratory, Golden, CO 80401; and dAcademia Sinica, Taipei City, Taiwan 115 Edited by Lonnie O. Ingram, University of Florida, Gainesville, FL, and approved September 23, 2016 (received for review April 6, 2016) Clostridium thermocellum can ferment cellulosic biomass to for- proposed pathway involving CO2 utilization in C. thermocellum is mate and other end products, including CO2. This organism lacks the malate shunt and its variant pathway (7, 14), in which CO2 is formate dehydrogenase (Fdh), which catalyzes the reduction of first incorporated by phosphoenolpyruvate carboxykinase (PEPCK) 13 CO2 to formate. However, feeding the bacterium C-bicarbonate to form oxaloacetate and then is released either by malic enzyme or and cellobiose followed by NMR analysis showed the production via oxaloacetate decarboxylase complex, yielding pyruvate. How- of 13C-formate in C. thermocellum culture, indicating the presence ever, other pathways capable of carbon fixation may also exist but of an uncharacterized pathway capable of converting CO2 to for- remain uncharacterized. mate. Combining genomic and experimental data, we demon- In recent years, isotopic tracer experiments followed by mass strated that the conversion of CO2 to formate serves as a CO2 spectrometry (MS) (15, 16) or NMR measurements (17) have entry point into the reductive one-carbon (C1) metabolism, and emerged as powerful tools for metabolic analysis. -
B.-Gioli -Co2-Fluxes-In-Florance.Pdf
Environmental Pollution 164 (2012) 125e131 Contents lists available at SciVerse ScienceDirect Environmental Pollution journal homepage: www.elsevier.com/locate/envpol Methane and carbon dioxide fluxes and source partitioning in urban areas: The case study of Florence, Italy B. Gioli a,*, P. Toscano a, E. Lugato a, A. Matese a, F. Miglietta a,b, A. Zaldei a, F.P. Vaccari a a Institute of Biometeorology (IBIMET e CNR), Via G.Caproni 8, 50145 Firenze, Italy b FoxLab, Edmund Mach Foundation, Via E. Mach 1, San Michele all’Adige, Trento, Italy article info abstract Article history: Long-term fluxes of CO2, and combined short-term fluxes of CH4 and CO2 were measured with the eddy Received 15 September 2011 covariance technique in the city centre of Florence. CO2 long-term weekly fluxes exhibit a high Received in revised form seasonality, ranging from 39 to 172% of the mean annual value in summer and winter respectively, while 12 January 2012 CH fluxes are relevant and don’t exhibit temporal variability. Contribution of road traffic and domestic Accepted 14 January 2012 4 heating has been estimated through multi-regression models combined with inventorial traffic and CH4 consumption data, revealing that heating accounts for more than 80% of observed CO2 fluxes. Those two Keywords: components are instead responsible for only 14% of observed CH4 fluxes, while the major residual part is Eddy covariance fl Methane fluxes likely dominated by gas network leakages. CH4 uxes expressed as CO2 equivalent represent about 8% of Gas network leakages CO2 emissions, ranging from 16% in summer to 4% in winter, and cannot therefore be neglected when assessing greenhouse impact of cities. -
Ecological and Evolutionary Effects of Interspecific Competition in Tits
Wilson Bull., 101(2), 1989, pp. 198-216 ECOLOGICAL AND EVOLUTIONARY EFFECTS OF INTERSPECIFIC COMPETITION IN TITS ANDRE A. DHONDT' AmTRAcT.-In this review the evidence for the existence of interspecific competition between members of the genus Purus is organized according to the time scale involved. Competition on an ecological time scale is amenable to experimental manipulation, whereas the effects of competition on an evolutionary time scale are not, Therefore the existence of competition has to be inferred mainly from comparisons between populations. Numerical effects of interspecific competition in coexisting populations on population parameters have been shown in several studies of Great and Blue tits (Parus major and P. caerulm) during the breeding season and during winter, and they have been suggested for the Black-capped Chickadee (P. atricapillus)and the Tufted Titmouse (P. bicolor).It is argued that the doubly asymmetric two-way interspecific competition between Great and Blue tits would have a stabilizing effect promoting their coexistence. Functional effects on niche use have been experimentally shown by removal or cage experiments between Willow (P. montanus)and Marsh (P. pahstris) tits, between Willow and Crested tits (P. cristatus)and Coal Tits (P. ater) and Goldcrests (Regulus reguh), and between Coal and Willow tits. Non-manipulative studies suggestthe existence of interspecific competition leading to rapid niche shifts between Crested and Willow tits and between Great and Willow tits. Evolutionary responses that can be explained as adaptations to variations in the importance of interspecific competition are numerous. An experiment failed to show that Blue Tit populations, subjected to different levels of interspecific competition by Great Tits, underwent divergent micro-evolutionary changes for body size. -
Modeling Biogeochemical Cycles
4 Modeling Biogeochemical Cycles Henning Rodhe 4.1 Introductory Remarks spatial average (i.e., the physical size of the reservoir itself) often has a horizontal size To formulate a model is to put together pieces of approaching that of a continent, or larger, i.e., knowledge about a particular system into a > 1000 km. The time scales corresponding to this consistent pattern that can form the basis for (1) spatial average are months, or longer. This interpretation of the past history of the system means that day-to-day variations in weather and (2) prediction of the future of the system. To and ocean currents are not generally considered be credible and useful, any model of a physical, explicitly when modeling biogeochemical cycles. chemical or biological system must rely on both The advent of fast computers and the avail- scientific fundamentals and observations of the ability of detailed data on the occurrence of world around us. High-quality observational certain chemical species have made it possible data are the basis upon which our understand- to construct meaningful cycle models with a ing of the environment rests. However, observa- much smaller and faster spatial and temporal tions themselves are not very useful unless the resolution. These spatial and time scales corre- results can be interpreted in some kind of model. spond to those in weather forecast models, i.e. Thus observations and modeling go hand in down to 100 km and 1 h. Transport processes hand. (e.g., for CO2 and sulfur compounds) in the This chapter focuses on types of models used oceans and atmosphere can be explicitly to describe the functioning of biogeochemical described in such models. -
Guidance for Identification and Naming of Substance Under REACH
Guidance for identification and naming of substances under 3 REACH and CLP Version 2.1 - May 2017 GUIDANCE Guidance for identification and naming of substances under REACH and CLP May 2017 Version 2.1 2 Guidance for identification and naming of substances under REACH and CLP Version 2.1 - May 2017 LEGAL NOTICE This document aims to assist users in complying with their obligations under the REACH and CLP regulations. However, users are reminded that the text of the REACH and CLP Regulations is the only authentic legal reference and that the information in this document does not constitute legal advice. Usage of the information remains under the sole responsibility of the user. The European Chemicals Agency does not accept any liability with regard to the use that may be made of the information contained in this document. Guidance for identification and naming of substances under REACH and CLP Reference: ECHA-16-B-37.1-EN Cat. Number: ED-07-18-147-EN-N ISBN: 978-92-9495-711-5 DOI: 10.2823/538683 Publ.date: May 2017 Language: EN © European Chemicals Agency, 2017 If you have any comments in relation to this document please send them (indicating the document reference, issue date, chapter and/or page of the document to which your comment refers) using the Guidance feedback form. The feedback form can be accessed via the EVHA Guidance website or directly via the following link: https://comments.echa.europa.eu/comments_cms/FeedbackGuidance.aspx European Chemicals Agency Mailing address: P.O. Box 400, FI-00121 Helsinki, Finland Visiting address: Annankatu 18, Helsinki, Finland Guidance for identification and naming of substances under 3 REACH and CLP Version 2.1 - May 2017 PREFACE This document describes how to name and identify a substance under REACH and CLP. -
Constructing and Analyzing Biological Interaction Networks for Knowledge Discovery
Constructing and Analyzing Biological Interaction Networks for Knowledge Discovery Dissertation Presented in Partial Fulfillment of the Requirements for the Degree Doctor of Philosophy in the Graduate School of The Ohio State University By Duygu Ucar Graduate Program in Computer Science and Engineering The Ohio State University 2009 Dissertation Committee: Srinivasan Parthasarathy, Advisor Yusu Wang Umit Catalyurek c Copyright by Duygu Ucar 2009 ABSTRACT Many biological datasets can be effectively modeled as interaction networks where nodes represent biological entities of interest such as proteins, genes, or complexes and edges mimic associations among them. The study of these biological network structures can provide insight into many biological questions including the functional characterization of genes and gene products, the characterization of DNA-protein bindings, and the under- standing of regulatory mechanisms. Therefore, the task of constructing biological interac- tion networks from raw data sets and exploiting information from these networks is critical, but is also fraught with challenges. First, the network structure is not always known in a priori; the structure should be inferred from raw and heterogeneous biological data sources. Second, biological networks are noisy (containing unreliable interactions) and incomplete (missing real interactions) which makes the task of extracting useful information difficult. Third, typically these networks have non-trivial topological properties (e.g., uneven degree distribution, small world) that limit the effectiveness of traditional knowledge discovery al- gorithms. Fourth, these networks are usually dynamic and investigation of their dynamics is essential to understand the underlying biological system. In this thesis, we address these issues by presenting a set of computational techniques that we developed to construct and analyze three specific types of biological interaction networks: protein-protein interaction networks, gene co-expression networks, and regulatory networks. -
Symbiotic Relationship in Which One Organism Benefits and the Other Is Unaffected
Ecology Quiz Review – ANSWERS! 1. Commensalism – symbiotic relationship in which one organism benefits and the other is unaffected. Mutualism – symbiotic relationship in which both organisms benefit. Parasitism – symbiotic relationship in which one organism benefits and the other is harmed or killed. Predation – a biological interaction where a predator feeds on a prey. 2A. Commensalism B. Mutualism C. Parasitism D. Predation 3. Autotrophic organisms produce their own food by way of photosynthesis. They are also at the base of a food chain or trophic pyramid. 4. Answer will vary – You will need two plants, two herbivores, and two carnivores 5. Decreases. Only 10% of the energy available at one level is passed to the next level. 6. If 10,000 units of energy are available to the grass at the bottom of the food chain, only 1000 units of energy will be available to the primary consumer, and only 100 units will be available to the secondary consumer. 7. Population is the number of a specific species living in an area. 8. B – All members of the Turdis migratorius species. 9. The number of secondary consumers would increase. 10. The energy decreases as you move up the pyramid which is indicated by the pyramid becoming smaller near the top. 11. Second highest-level consumer would increase. 12. Primary consumer would decrease due to higher numbers of secondary consumer. 13. The number of organisms would decrease due to the lack of food for primary consumers. Other consumers would decrease as the numbers of their food source declined. 14. Number of highest-level consumers would decrease due to lack of food. -
Guide to Best Practices for Ocean CO2 Measurements
Guide to Best Practices for Ocean C0 for to Best Practices Guide 2 probe Measurments to digital thermometer thermometer probe to e.m.f. measuring HCl/NaCl system titrant burette out to thermostat bath combination electrode In from thermostat bath mL Metrohm Dosimat STIR Guide to Best Practices for 2007 This Guide contains the most up-to-date information available on the Ocean CO2 Measurements chemistry of CO2 in sea water and the methodology of determining carbon system parameters, and is an attempt to serve as a clear and PICES SPECIAL PUBLICATION 3 unambiguous set of instructions to investigators who are setting up to SPECIAL PUBLICATION PICES IOCCP REPORT No. 8 analyze these parameters in sea water. North Pacific Marine Science Organization 3 PICES SP3_Cover.indd 1 12/13/07 4:16:07 PM Publisher Citation Instructions North Pacific Marine Science Organization Dickson, A.G., Sabine, C.L. and Christian, J.R. (Eds.) 2007. P.O. Box 6000 Guide to Best Practices for Ocean CO2 Measurements. Sidney, BC V8L 4B2 PICES Special Publication 3, 191 pp. Canada www.pices.int Graphic Design Cover and Tabs Caveat alkemi creative This report was developed under the guidance of the Victoria, BC, Canada PICES Science Board and its Section on Carbon and climate. The views expressed in this report are those of participating scientists under their responsibilities. ISSN: 1813-8519 ISBN: 1-897176-07-4 This book is printed on FSC certified paper. The cover contains 10% post-consumer recycled content. PICES SP3_Cover.indd 2 12/13/07 4:16:11 PM Guide to Best Practices for Ocean CO2 Measurements PICES SPECIAL PUBLICATION 3 IOCCP REPORT No. -
When the Air Turns the Oceans Sour
2200 pH value 8.3 8.2 8.1 8.0 7.9 7.8 7.7 7.6 7.5 7.4 7.3 7.2 7.1 Unfavorable prognosis: According to simulations of researchers at the Max Planck Institute in Hamburg, the pH value of the oceanic surface layer will be considerably lower in 2200 than in 1950, visible in the color shift within the area shown in red (left). The oceans are becoming more acidic. FOCUS_Geosciences When the Air Turns the Oceans Sour Human society has begun an ominous large-scale experiment, the full consequences of which will not be foreseeable for some time yet. Massive emissions of man-made carbon dioxide are heating up the Earth. But that’s not all: the increased concentration of this greenhouse gas in the atmosphere is also acidifying the oceans. Tatiana Ilyina and her staff at the Max Planck Institute for Meteorology in Hamburg are researching the consequences this could have. TEXT TIM SCHRÖDER hey’re called “sea butterflies” are warming the Earth like a green- because they float in the house. Less well known is the fact that ocean like a small winged the rising concentration of carbon diox- creature. However, pteropods ide in the atmosphere also leads to the belong to the gastropod class oceans slowly becoming more acidic. T of mollusks. They paddle through the This is because the oceans absorb a large water with shells as small as a baby’s portion of the carbon dioxide from the fingernail, and strangely transparent atmosphere. Put simply, the gas forms skin. -
A Regional Study
POPULATION STRUCTURE AND INTERREGIONAL INTERACTION IN PRE- HISPANIC MESOAMERICA: A BIODISTANCE STUDY DISSERTATION Presented in Partial Fulfillment of the Requirements for the Degree Doctor of Philosophy in the Graduate School of the Ohio State University By B. Scott Aubry, B.A., M.A. ***** The Ohio State University 2009 Dissertation Committee: Approved by Professor Clark Spencer Larsen, Adviser Professor Paul Sciulli _________________________________ Adviser Professor Sam Stout Graduate Program in Anthropology Professor Robert DePhilip Copyright Bryan Scott Aubry 2009 ABSTRACT This study addresses long standing issues regarding the nature of interregional interaction between central Mexico and the Maya area through the analysis of dental variation. In total 25 sites were included in this study, from Teotihuacan and Tula, to Tikal and Chichen Itza. Many other sites were included in this study to obtain a more comprehensive picture of the biological relationships between these regions and to better estimate genetic heterozygosity for each sub-region. The scope of the present study results in a more comprehensive understanding of population interaction both within and between the sub-regions of Mesoamerica, and it allows for the assessment of differential interaction between sites on a regional scale. Both metric and non-metric data were recorded. Non-metric traits were scored according to the ASU system, and dental metrics include the mesiodistal and buccolingual dimensions at the CEJ following a modification of Hillson et al. (2005). Biodistance estimates were calculated for non-metric traits using Mean Measure of Divergence. R-matrix analysis, which provides an estimate of average genetic heterozygosity, was applied to the metric data. R-matrix analysis was performed for each of the sub-regions separately in order to detect specific sites that deviate from expected levels of genetic heterozygosity in each area. -
Study of the System Cao-Sio2-H2O at 30 C and of the Reaction of Water On
U.S. Department of Commerce Bureau of Standards RESEARCH PAPER RP687 Part of Bureau of Standards Journal of Research, vol. 12, June 1934 STUDY OF THE SYSTEM CaO-Si02-H2 AT 30 C AND OF THE REACTION OF WATER ON THE ANHYDROUS CALCIUM SILICATES By E. P. Flint and Lansing S. Wells abstract A graph has been prepared representing the solubility of silica at 30 C in solu- tions containing increasing concentrations of lime up to saturation, the solutions being in "equilibrium" with solid phases composed of hydrated combinations of lime and silica. An electrometric and analytical study of both supersaturated and unsaturated solutions of silica at definite concentrations of lime, when corre- lated with these equilibrium solubility relationships, indicates the existence of definite compounds in the system CaO-Si0 2-H 2 which may be the hydrated calcium salts of orthosilicic acid. Experimental values were obtained for the hydrolysis constants of these salts from which ionization constants for the four steps in the dissociation of orthosilicic acid were calculated. A study of the reaction of water upon the anhydrous calcium silicates (CaO Si02 , 3CaO- 2Si0 2 , 7-2CaO-Si0 ,3-2CaO Si0 , ) showed that the products formed depend 2 , 2 3CaOSi0 2 upon the equilibrium relationships of the system CaO-Si02-H 2 0. Of these compounds all but monocalcium silicate (CaO-Si02 ) form solutions which pre- cipitate hydrated calcium silicate on standing. Application of the same methods to a study of the reaction of water upon a portland cement indicated that hydrated calcium orthosilicate is formed during the hydration of portland cement.