Comparison Between Highly Elliptical Orbit and Medium Earth Orbit Constellations
Total Page:16
File Type:pdf, Size:1020Kb
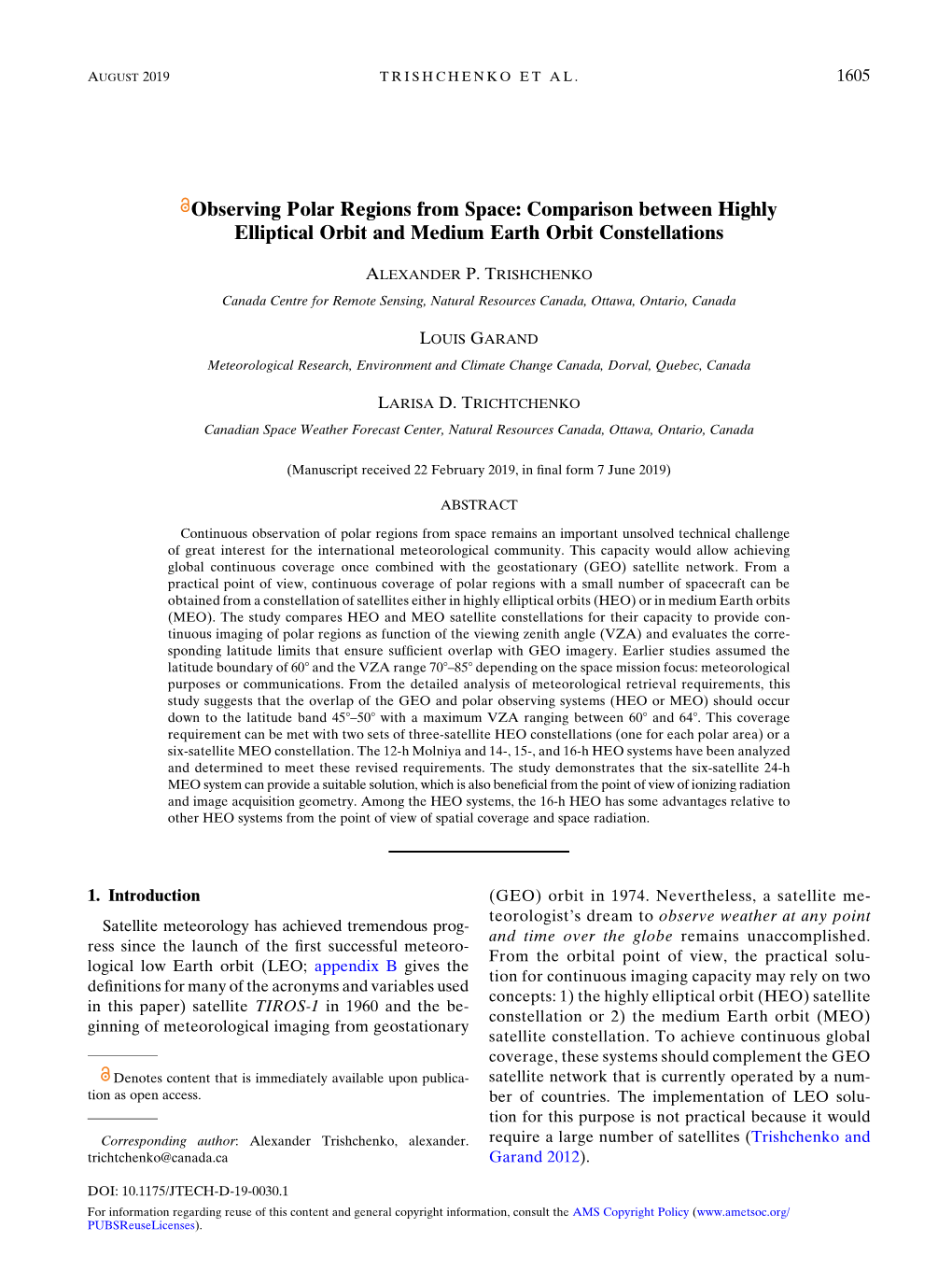
Load more
Recommended publications
-
Analysis of Perturbations and Station-Keeping Requirements in Highly-Inclined Geosynchronous Orbits
ANALYSIS OF PERTURBATIONS AND STATION-KEEPING REQUIREMENTS IN HIGHLY-INCLINED GEOSYNCHRONOUS ORBITS Elena Fantino(1), Roberto Flores(2), Alessio Di Salvo(3), and Marilena Di Carlo(4) (1)Space Studies Institute of Catalonia (IEEC), Polytechnic University of Catalonia (UPC), E.T.S.E.I.A.T., Colom 11, 08222 Terrassa (Spain), [email protected] (2)International Center for Numerical Methods in Engineering (CIMNE), Polytechnic University of Catalonia (UPC), Building C1, Campus Norte, UPC, Gran Capitan,´ s/n, 08034 Barcelona (Spain) (3)NEXT Ingegneria dei Sistemi S.p.A., Space Innovation System Unit, Via A. Noale 345/b, 00155 Roma (Italy), [email protected] (4)Department of Mechanical and Aerospace Engineering, University of Strathclyde, 75 Montrose Street, Glasgow G1 1XJ (United Kingdom), [email protected] Abstract: There is a demand for communications services at high latitudes that is not well served by conventional geostationary satellites. Alternatives using low-altitude orbits require too large constellations. Other options are the Molniya and Tundra families (critically-inclined, eccentric orbits with the apogee at high latitudes). In this work we have considered derivatives of the Tundra type with different inclinations and eccentricities. By means of a high-precision model of the terrestrial gravity field and the most relevant environmental perturbations, we have studied the evolution of these orbits during a period of two years. The effects of the different perturbations on the constellation ground track (which is more important for coverage than the orbital elements themselves) have been identified. We show that, in order to maintain the ground track unchanged, the most important parameters are the orbital period and the argument of the perigee. -
A Highly Elliptical Orbit Space System for Hydrometeorological Monitoring of the Arctic Region by V
A highly elliptical orbit space system for hydrometeorological monitoring of the Arctic region by V. V. Asmus1, V. N. Dyadyuchenko2, Y. I. Nosenko3, G. M. Polishchuk4 and V. A. Selin3 The lack of reliable, frequently high latitudes. It has therefore been • Monitoring of climate change updated information on the Earth’s suggested that demonstration of polar ice caps is a signifi cant problem a hydrometeorological system of • Data collection and relay for weather forecasting, affecting satellites on highly elliptical orbit from land-, sea- and air-based forecast skill for the entire planet. The (HEO), called the “Arctica” system, observing platforms poor numerical weather prediction should be created to provide the (NWP) skill for the Arctic region necessary complex information for the • Exchange and dissemination of and the Earth’s northern territories diffi cult tasks involved in developing processed hydrometeorological is caused primarily by errors in the whole Arctic region. and heliogeophysical data. determining initial conditions, which depend on the quality of initial Signifi cantly, the hydrometeorological Further progress in global and data. Until now, initial data have observations carried out in the regional numerical weather prediction been received from meteorological Arctic within the framework of the depends to a large extent on: geostationary satellites, which are International Polar Year 2007-2008 not very effective in scanning high are not provided with remote-sensing • Quasi-continuous reception latitudes and polar-orbiting -
GPS Applications in Space
Space Situational Awareness 2015: GPS Applications in Space James J. Miller, Deputy Director Policy & Strategic Communications Division May 13, 2015 GPS Extends the Reach of NASA Networks to Enable New Space Ops, Science, and Exploration Apps GPS Relative Navigation is used for Rendezvous to ISS GPS PNT Services Enable: • Attitude Determination: Use of GPS enables some missions to meet their attitude determination requirements, such as ISS • Real-time On-Board Navigation: Enables new methods of spaceflight ops such as rendezvous & docking, station- keeping, precision formation flying, and GEO satellite servicing • Earth Sciences: GPS used as a remote sensing tool supports atmospheric and ionospheric sciences, geodesy, and geodynamics -- from monitoring sea levels and ice melt to measuring the gravity field ESA ATV 1st mission JAXA’s HTV 1st mission Commercial Cargo Resupply to ISS in 2008 to ISS in 2009 (Space-X & Cygnus), 2012+ 2 Growing GPS Uses in Space: Space Operations & Science • NASA strategic navigation requirements for science and 20-Year Worldwide Space Mission space ops continue to grow, especially as higher Projections by Orbit Type* precisions are needed for more complex operations in all space domains 1% 5% Low Earth Orbit • Nearly 60%* of projected worldwide space missions 27% Medium Earth Orbit over the next 20 years will operate in LEO 59% GeoSynchronous Orbit – That is, inside the Terrestrial Service Volume (TSV) 8% Highly Elliptical Orbit Cislunar / Interplanetary • An additional 35%* of these space missions that will operate at higher altitudes will remain at or below GEO – That is, inside the GPS/GNSS Space Service Volume (SSV) Highly Elliptical Orbits**: • In summary, approximately 95% of projected Example: NASA MMS 4- worldwide space missions over the next 20 years will satellite constellation. -
NASA Process for Limiting Orbital Debris
NASA-HANDBOOK NASA HANDBOOK 8719.14 National Aeronautics and Space Administration Approved: 2008-07-30 Washington, DC 20546 Expiration Date: 2013-07-30 HANDBOOK FOR LIMITING ORBITAL DEBRIS Measurement System Identification: Metric APPROVED FOR PUBLIC RELEASE – DISTRIBUTION IS UNLIMITED NASA-Handbook 8719.14 This page intentionally left blank. Page 2 of 174 NASA-Handbook 8719.14 DOCUMENT HISTORY LOG Status Document Approval Date Description Revision Baseline 2008-07-30 Initial Release Page 3 of 174 NASA-Handbook 8719.14 This page intentionally left blank. Page 4 of 174 NASA-Handbook 8719.14 This page intentionally left blank. Page 6 of 174 NASA-Handbook 8719.14 TABLE OF CONTENTS 1 SCOPE...........................................................................................................................13 1.1 Purpose................................................................................................................................ 13 1.2 Applicability ....................................................................................................................... 13 2 APPLICABLE AND REFERENCE DOCUMENTS................................................14 3 ACRONYMS AND DEFINITIONS ...........................................................................15 3.1 Acronyms............................................................................................................................ 15 3.2 Definitions ......................................................................................................................... -
Arxiv:2103.06251V2 [Nlin.CD] 24 May 2021 Resonant Terms Are Algebraically Computed up to the 4Th Degree and Order
DYNAMICAL PROPERTIES OF THE MOLNIYA SATELLITE CONSTELLATION: LONG-TERM EVOLUTION OF THE SEMI-MAJOR AXIS J. DAQUIN, E.M. ALESSI, J. O'LEARY, A. LEMAITRE, AND A. BUZZONI Abstract. We describe the phase space structures related to the semi-major axis of Molniya-like satellites subject to tesseral and lunisolar resonances. In particular, the questions answered in this contribution are: (i) we study the indirect interplay of the critical inclination resonance on the semi-geosynchronous resonance using a hierarchy of more realistic dynamical systems, thus discussing the dynamics beyond the integrable approximation. By introducing ad hoc tractable models averaged over fast angles, (ii) we numerically demarcate the hyperbolic structures organising the long-term dynamics via Fast Lyapunov Indicators cartography. Based on the publicly available two-line elements space orbital data, (iii) we identify two satellites, namely Molniya 1-69 and Molniya 1-87, displaying fingerprints consistent with the dynamics associated to the hyperbolic set. Finally, (iv) the computations of their associated dynamical maps highlight that the spacecraft are trapped within the hyperbolic tangle. This research therefore reports evidence of actual artificial satellites in the near-Earth environment whose dynamics are ruled by manifolds and resonant mechanisms. The tools, formalism and methodologies we present are exportable to other region of space subject to similar commensurabilities as the geosynchronous region. 1. Introduction The present manuscript is part of a recent series of papers [1, 6] dedicated to astrodynamical properties of Molniya spacecraft. It is well-known that the Molniya orbit provides a valuable dynamical alternative to the geosynchronous orbit, suitable for communication satellites to deliver a service in high-latitude countries, as it is actually the case for Russia. -
Open Rosen Thesis.Pdf
THE PENNSYLVANIA STATE UNIVERSITY SCHREYER HONORS COLLEGE DEPARTMENT OF AEROSPACE ENGINEERING END OF LIFE DISPOSAL OF SATELLITES IN HIGHLY ELLIPTICAL ORBITS MITCHELL ROSEN SPRING 2019 A thesis submitted in partial fulfillment of the requirements for a baccalaureate degree in Aerospace Engineering with honors in Aerospace Engineering Reviewed and approved* by the following: Dr. David Spencer Professor of Aerospace Engineering Thesis Supervisor Dr. Mark Maughmer Professor of Aerospace Engineering Honors Adviser * Signatures are on file in the Schreyer Honors College. i ABSTRACT Highly elliptical orbits allow for coverage of large parts of the Earth through a single satellite, simplifying communications in the globe’s northern reaches. These orbits are able to avoid drastic changes to the argument of periapse by using a critical inclination (63.4°) that cancels out the first level of the geopotential forces. However, this allows the next level of geopotential forces to take over, quickly de-orbiting satellites. Thus, a balance between the rate of change of the argument of periapse and the lifetime of the orbit is necessitated. This thesis sets out to find that balance. It is determined that an orbit with an inclination of 62.5° strikes that balance best. While this orbit is optimal off of the critical inclination, it is still near enough that to allow for potential use of inclination changes as a deorbiting method. Satellites are deorbited when the propellant remaining is enough to perform such a maneuver, and nothing more; therefore, the less change in velocity necessary for to deorbit, the better. Following the determination of an ideal highly elliptical orbit, the different methods of inclination change is tested against the usual method for deorbiting a satellite, an apoapse burn to lower the periapse, to find the most propellant- efficient method. -
GEO, MEO, and LEO How Orbital Altitude Impacts Network Performance in Satellite Data Services
GEO, MEO, AND LEO How orbital altitude impacts network performance in satellite data services COMMUNICATION OVER SATELLITE “An entire multi-orbit is now well accepted as a key enabler across the telecommunications industry. Satellite networks can supplement existing infrastructure by providing global reach satellite ecosystem is where terrestrial networks are unavailable or not feasible. opening up above us, But not all satellite networks are created equal. Providers offer different solutions driving new opportunities depending on the orbits available to them, and so understanding how the distance for high-performance from Earth affects performance is crucial for decision making when selecting a satellite service. The following pages give an overview of the three main orbit gigabit connectivity and classes, along with some of the principal trade-offs between them. broadband services.” Stewart Sanders Executive Vice President of Key terms Technology at SES GEO – Geostationary Earth Orbit. NGSO – Non-Geostationary Orbit. NGSO is divided into MEO and LEO. MEO – Medium Earth Orbit. LEO – Low Earth Orbit. HTS – High Throughput Satellites designed for communication. Latency – the delay in data transmission from one communication endpoint to another. Latency-critical applications include video conferencing, mobile data backhaul, and cloud-based business collaboration tools. SD-WAN – Software-Defined Wide Area Networking. Based on policies controlled by the user, SD-WAN optimises network performance by steering application traffic over the most suitable access technology and with the appropriate Quality of Service (QoS). Figure 1: Schematic of orbital altitudes and coverage areas GEOSTATIONARY EARTH ORBIT Altitude 36,000km GEO satellites match the rotation of the Earth as they travel, and so remain above the same point on the ground. -
Orbital Debris Mitigation Standard Practices (ODMSP) Were Established in 2001 to Address the Increase in Orbital Debris in the Near-Earth Space Environment
U.S. Government Orbital Debris Mitigation Standard Practices, November 2019 Update PREAMBLE The United States Government (USG) Orbital Debris Mitigation Standard Practices (ODMSP) were established in 2001 to address the increase in orbital debris in the near-Earth space environment. The goal of the ODMSP was to limit the generation of new, long-lived debris by the control of debris released during normal operations, minimizing debris generated by accidental explosions, the selection of safe flight profile and operational configuration to minimize accidental collisions, and postmission disposal of space structures. While the original ODMSP adequately protected the space environment at the time, the USG recognizes that it is in the interest of all nations to minimize new debris and mitigate effects of existing debris. This fact, along with increasing numbers of space missions, highlights the need to update the ODMSP and to establish standards that can inform development of international practices. This 2019 update includes improvements to the original objectives as well as clarification and additional standard practices for certain classes of space operations. The improvements consist of a quantitative limit on debris released during normal operations, a probability limit on accidental explosions, probability limits on accidental collisions with large and small debris, and a reliability threshold for successful postmission disposal. The new standard practices established in the update include the preferred disposal options for immediate removal of structures from the near-Earth space environment, a low-risk geosynchronous Earth orbit (GEO) transfer disposal option, a long-term reentry option, and improved move-away-and-stay-away storage options in medium Earth orbit (MEO) and above GEO. -
SATELLITES ORBIT ELEMENTS : EPHEMERIS, Keplerian ELEMENTS, STATE VECTORS
www.myreaders.info www.myreaders.info Return to Website SATELLITES ORBIT ELEMENTS : EPHEMERIS, Keplerian ELEMENTS, STATE VECTORS RC Chakraborty (Retd), Former Director, DRDO, Delhi & Visiting Professor, JUET, Guna, www.myreaders.info, [email protected], www.myreaders.info/html/orbital_mechanics.html, Revised Dec. 16, 2015 (This is Sec. 5, pp 164 - 192, of Orbital Mechanics - Model & Simulation Software (OM-MSS), Sec 1 to 10, pp 1 - 402.) OM-MSS Page 164 OM-MSS Section - 5 -------------------------------------------------------------------------------------------------------43 www.myreaders.info SATELLITES ORBIT ELEMENTS : EPHEMERIS, Keplerian ELEMENTS, STATE VECTORS Satellite Ephemeris is Expressed either by 'Keplerian elements' or by 'State Vectors', that uniquely identify a specific orbit. A satellite is an object that moves around a larger object. Thousands of Satellites launched into orbit around Earth. First, look into the Preliminaries about 'Satellite Orbit', before moving to Satellite Ephemeris data and conversion utilities of the OM-MSS software. (a) Satellite : An artificial object, intentionally placed into orbit. Thousands of Satellites have been launched into orbit around Earth. A few Satellites called Space Probes have been placed into orbit around Moon, Mercury, Venus, Mars, Jupiter, Saturn, etc. The Motion of a Satellite is a direct consequence of the Gravity of a body (earth), around which the satellite travels without any propulsion. The Moon is the Earth's only natural Satellite, moves around Earth in the same kind of orbit. (b) Earth Gravity and Satellite Motion : As satellite move around Earth, it is pulled in by the gravitational force (centripetal) of the Earth. Contrary to this pull, the rotating motion of satellite around Earth has an associated force (centrifugal) which pushes it away from the Earth. -
Nonlinear Filtering for Autonomous Navigation of Spacecraft in Highly Elliptical Orbit
Nonlinear Filtering for Autonomous Navigation of Spacecraft in Highly Elliptical Orbit by Adam C. Vigneron, B.Sc.(Eng.) A thesis submitted to the Faculty of Graduate and Postdoctoral Affairs in partial fulfillment of the requirements for the degree of Master of Applied Science Ottawa-Carleton Institute for Mechanical and Aerospace Engineering Department of Mechanical and Aerospace Engineering Carleton University Ottawa, Ontario May, 2014 c Copyright Adam C. Vigneron, 2014 The undersigned hereby recommends to the Faculty of Graduate and Postdoctoral Affairs acceptance of the thesis Nonlinear Filtering for Autonomous Navigation of Spacecraft in Highly Elliptical Orbit submitted by Adam C. Vigneron, B.Sc.(Eng.) in partial fulfillment of the requirements for the degree of Master of Applied Science Professor Anton H. J. de Ruiter, Thesis Supervisor Professor Bruce Burlton, Thesis Co-supervisor Professor Alex Ellery, Thesis Co-supervisor Professor Metin Yaras, Chair, Department of Mechanical and Aerospace Engineering Ottawa-Carleton Institute for Mechanical and Aerospace Engineering Department of Mechanical and Aerospace Engineering Carleton University May, 2014 ii Abstract To fill a gap in satellite services for the Canadian Arctic, the Canadian Space Agency has proposed a Polar Communication and Weather (PCW) mission to be flown in a highly elliptical Molniya orbit. In an era of increasingly capable space hardware, au- tonomous satellite navigation has become a standard means by which satellites in low Earth orbit can increase their independence and functionality. This study examined the accuracy to which autonomous navigation might be realized in a Molniya orbit. Using appropriate physical force models and simulated pseudorange signals from the Global Positioning System (GPS), a navigation algorithm based on the Extended Kalman Filter was demonstrated to achieve a three-dimensional root-mean-square accuracy of 58:9 m over a 500 km ¢ 40 000 km Molniya orbit. -
Signal in Space Error and Ephemeris Validity Time Evaluation of Milena and Doresa Galileo Satellites †
sensors Article Signal in Space Error and Ephemeris Validity Time Evaluation of Milena and Doresa Galileo Satellites † Umberto Robustelli * , Guido Benassai and Giovanni Pugliano Department of Engineering, Parthenope University of Naples, 80143 Napoli, Italy; [email protected] (G.B.); [email protected] (G.P.) * Correspondence: [email protected] † This paper is an extended version of our paper published in the 2018 IEEE International Workshop on Metrology for the Sea proceedings entitled “Accuracy evaluation of Doresa and Milena Galileo satellites broadcast ephemeris”. Received: 14 March 2019; Accepted: 11 April 2019; Published: 14 April 2019 Abstract: In August 2016, Milena (E14) and Doresa (E18) satellites started to broadcast ephemeris in navigation message for testing purposes. If these satellites could be used, an improvement in the position accuracy would be achieved. A small error in the ephemeris would impact the accuracy of positioning up to ±2.5 m, thus orbit error must be assessed. The ephemeris quality was evaluated by calculating the SISEorbit (in orbit Signal In Space Error) using six different ephemeris validity time thresholds (14,400 s, 10,800 s, 7200 s, 3600 s, 1800 s, and 900 s). Two different periods of 2018 were analyzed by using IGS products: DOYs 52–71 and DOYs 172–191. For the first period, two different types of ephemeris were used: those received in IGS YEL2 station and the BRDM ones. Milena (E14) and Doresa (E18) satellites show a higher SISEorbit than the others. If validity time is reduced, the SISEorbit RMS of Milena (E14) and Doresa (E18) greatly decreases differently from the other satellites, for which the improvement, although present, is small. -
Broadcast Ephemeris Model of the Beidou Navigation Satellite System
JOURNAL OF Journal of Engineering Science and Technology Review 10 (4) (2017) 65-71 Engineering Science and estr Technology Review J Research Article www.jestr.org Broadcast Ephemeris Model of the BeiDou Navigation Satellite System Xie Xiaogang* and Lu Mingquan Department of Electronic Engineering, Tsinghua University, Beijing 100084, China Received 2 March 2017; Accepted 1 August 2017 ___________________________________________________________________________________________ Abstract The BeiDou Navigation Satellite System (BDS) space constellation is composed of geostationary earth orbit (GEO), medium earth orbit (MEO), and inclined geosynchronous satellite orbit (IGSO) satellites, all of which adopt the Global Positioning System (GPS) broadcast ephemeris model of the United States of America (U.S.A). However, in the case of small eccentricity and small orbit inclination angle, the coefficient matrix is non-positive when fitting the broadcast ephemeris parameters due to the fuzzy definition of a few Keplerian orbit elements, thereby resulting in low precision or even failure. This study proposed a novel broadcast ephemeris model based on the second class of non-singular orbit elements. The proposed model can address the unsuitability of the classical broadcast ephemeris model in the condition of small eccentricity and small orbit inclination angle, and unify the broadcast ephemeris parameters user algorithm models of GEO, MEO, and IGSO. The proposed model is a 14-parameters broadcast ephemeris model constructed with the second class of non-singular orbit elements and the corresponding broadcast ephemeris parameters perturbation over time. Lastly, the proposed model was verified by precision orbit data generated by the Satellite Tool Kit (STK). Results show that the 14-parameters broadcast ephemeris model is suitable for the GEO, MEO, and IGSO satellites and has high fitting precision to fully meet the requirements of the BDS.