In Situ XANES Study of the Influence of Varying Temperature and Oxygen
Total Page:16
File Type:pdf, Size:1020Kb
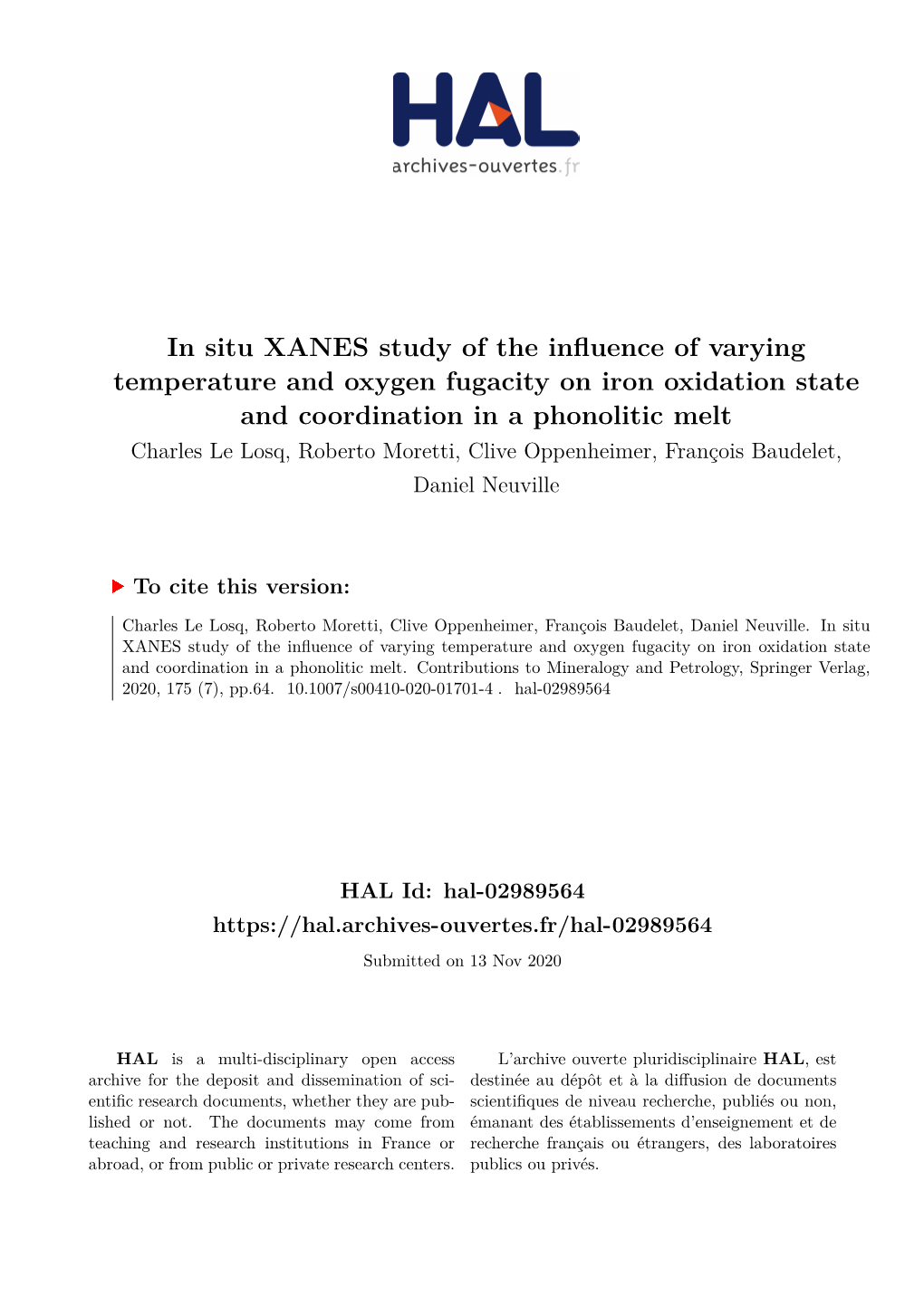
Load more
Recommended publications
-
Geophysical Monograph Series
Geophysical Monograph Series Including IUGG Volumes Maurice Ewing Volumes Mineral Physics Volumes Geophysical Monograph Series 163 Remote Sensing of Northern Hydrology: Measuring 181 Midlatitude Ionospheric Dynamics and Disturbances Environmental Change Claude R. Duguay and Alain Paul M. Kintner, Jr., Anthea J. Coster, Tim Fuller-Rowell, Pietroniro (Eds.) Anthony J. Mannucci, Michael Mendillo, and 164 Archean Geodynamics and Environments Keith Benn, Roderick Heelis (Eds.) Jean-Claude Mareschal, and Kent C. Condie (Eds.) 182 The Stromboli Volcano: An Integrated Study of 165 Solar Eruptions and Energetic Particles the 2002–2003 Eruption Sonia Calvari, Salvatore Natchimuthukonar Gopalswamy, Richard Mewaldt, Inguaggiato, Giuseppe Puglisi, Maurizio Ripepe, and Jarmo Torsti (Eds.) and Mauro Rosi (Eds.) 166 Back-Arc Spreading Systems: Geological, Biological, 183 Carbon Sequestration and Its Role in the Global Chemical, and Physical Interactions David M. Christie, Carbon Cycle Brian J. McPherson and Charles Fisher, Sang-Mook Lee, and Sharon Givens (Eds.) Eric T. Sundquist (Eds.) 167 Recurrent Magnetic Storms: Corotating Solar 184 Carbon Cycling in Northern Peatlands Andrew J. Baird, Wind Streams Bruce Tsurutani, Robert McPherron, Lisa R. Belyea, Xavier Comas, A. S. Reeve, and Walter Gonzalez, Gang Lu, José H. A. Sobral, and Lee D. Slater (Eds.) Natchimuthukonar Gopalswamy (Eds.) 185 Indian Ocean Biogeochemical Processes and 168 Earth’s Deep Water Cycle Steven D. Jacobsen and Ecological Variability Jerry D. Wiggert, Suzan van der Lee (Eds.) Raleigh R. Hood, S. Wajih A. Naqvi, Kenneth H. Brink, 169 Magnetospheric ULF Waves: Synthesis and and Sharon L. Smith (Eds.) New Directions Kazue Takahashi, Peter J. Chi, 186 Amazonia and Global Change Michael Keller, Richard E. -
Challenges and Opportunities in Materials for Green Energy Production and Conversion
CONFERENCES VIRTUAL MEETING | 2021 15 - 17 June 2021 Challenges and opportunities in materials for green energy production and conversion LOCATION VIRTUAL MEETING CONVENORS Dr Edurne Serrano- Larrea FORMER LE STUDIUM RESEARCH FELLOW / ARD 2020 - LAVOISIER PROGRAMME Mineralogy and Petrology Department, UPV/ EHU - ES Dr Conchi Ania & Dr Encarnacion Raymundo-Piñero Extreme Conditions and Materials: High Temperature and Irradiation (CEMHTI) / CNRS - FR CONFERENCES VIRTUAL MEETING | 15-17 JUNE 2021 ABSTRACTS Challenges and opportunities in materials for green energy production and conversion CONVENORS Dr Edurne Serrano-Larrea FORMER LE STUDIUM RESEARCH FELLOW / LAVOISIER ARD 2020 PROGRAMME FROM: Mineralogy and Petrology Department, UPV/EHU - ES Dr Conchi Ania Extreme Conditions and Materials: High Temperature and Irradiation (CEMHTI) / CNRS - FR Dr Encarnacion Raymundo-Piñero Extreme Conditions and Materials: High Temperature and Irradiation (CEMHTI) / CNRS - FR ORGANIZING COMMITTEE Sophie Gabillet, General Secretary Dr Aurélien Montagu, Scientific Relations Manager Maurine Villiers, Communication & Events Manager LE STUDIUM Loire Valley Institute for Advanced Studies • Région Centre-Val de Loire • FR LE STUDIUM CONFERENCES Challenges and opportunities in materials for green energy production and conversion |15-17 June 2021 |3 EDITO Created in 1996 on the CNRS campus in Orleans La Source, LE STUDIUM has Development 2020 programmes initiated by the Centre-Val de Loire Regional evolved to become the multidisciplinary Loire Valley Institute for Advanced Council to support the smart specialisation strategy (S3) around 5 main axes: Studies (IAS), operating in the Centre-Val de Loire region of France. LE biopharmaceuticals, renewable energies, cosmetics, environmental metrology STUDIUM has its headquarters in the city centre of Orleans in a newly renovated and natural and cultural heritage. -
Scott Polar Research Institute Review 2009 83Rd Annual Report of the Scott Polar Research Institute University of Cambridge, UK
Scott Polar Research Institute Review 2009 83rd Annual Report of the Scott Polar Research Institute University of Cambridge, UK Printed in Great Britain by ESL Studio Studio House, Invar Business Park, Invar Road, Swinton, Manchester M27 9HF Tel: 0161 793 7377 Detail of the façade of the Institute, with polar bear and fish Contents Director’s Introduction ....................................................................................................2 Institute Staff ....................................................................................................................4 Polar Research .................................................................................................6 Research Group Structure Polar Physical Science Polar Social Science and Humanities Current Research Grants Publications by Institute Staff .........................................................................14 Books Papers in Peer-Reviewed Journals Chapters in Books and Other Contributions Student Doctoral and Masters Theses Seminars Polar Information and Historic Archives ..........................................................16 Library and Information Service World Data Centre for Glaciology, Cambridge Picture Library Archives Polar Record SPRI Website Teaching, Learning and Understanding ..........................................................20 University Teaching SPRI Museum Projecting the Significance of the Polar Regions Expedition Support: Gino Watkins Funds External Contributions to Polar Activities ........................................................22 -
Annual Report
The Department of Geography Annual Report Picture by Prof Ulf Büntgen 2016-17 Contents Introduction 3 Staff Updates 4-5 Promotions, Honours and Prizes 5 Anthena Swan Award 5 Research 6-10 Key Grants Awarded 6 Research Features 7 Research Outreach 8 Books 2016-17 9 Postdoctoral Research Fellows 10 Departmental Seminars 10 Visiting Scholars 10 Distinguished International Visitors 10 Undergraduate Studies 11 Distinctions and Prizes 12 First Class Dissertations 13 Cambridge University Geographical Society 14 Graduate Studies 15 PhDs Submitted and Completed 16 Communications Updates 17 Technical Services 18 Staff 19 Tenerife Fieldtrip Annual Report 2016-17 2 Department of Geography Introduction Main Geography Building Ash Amin Head of Department 2016-17 has been a busy and productive year for the Department of Geography as we welcomed a clutch of new academic staff, following a large number of staff retirements the previous year. These new colleagues will push our teaching and research into fresh and exciting directions, and we bid them all a very warm welcome to Cambridge. It has been a year of achievements and awards: in June, total of £5,026,851 in research grants awarded, and Andrew Cliff became the eleventh Cambridge academic published over 154 monographs, articles, chapters and to win the Royal Geographical Society Victoria Medal, working papers. Our sub departments, the Scott Polar and in April Charlotte Lemanski received a Geographical Research Institute and the newly launched University Association Journal Article Award. We have also enjoyed of Cambridge Conservation Research Institute, both success in our forays into film. Matthew Gandy’s film continue to serve as centres of excellence within their Natura Urbana won ‘Best German Biodiversity Film’ at respective fields. -
Into the Inferno: Volcanology on Film with Clive Oppenheimer Forum
landmark Summer 2017 I Edition 5 Into the Inferno: Volcanology on film with Clive Oppenheimer Forum Theatre in an Age of Austerity: Mia Gray and Susan Smith explore Austerity Britain on stage The Department of Geography alumni magazine Inside Interview: James Blake 4 Into the Inferno 6 Forum Theatre in an Age of Austerity 8 Staff Profile: Matthew Gandy 10 Turtles of the Caribbean 12 News 14 Welcome to the new-look landmark fter a few years’ hiatus, it’s my pleasure to bring you the latest version of Landmark, the magazine for alumni of the Department of Geography at the University of Cambridge. Landmark will be coming to you annually, packed full of news on Department research, events A and alumni updates. We hope you enjoy it. Since the last edition of Landmark in 2010, Cambridge Geography has continued to excel, regularly featuring at the top of league tables in teaching and research. Our staff of 35 academics publish ground- breaking research, win prizes, and attract large grants from many different sectors. On pages 6–9 you can read about two of the Department’s forays into popular culture: Prof Clive Oppenheimer writes of his award-winning Netflix documentary Into the Inferno, while Dr Mia Gray and Prof Susan Smith reflect on their nationwide theatre tour, exploring the effects of austerity on modern Britain. We are heralding the start of a new era as we say goodbye to a cohort of professors who have been with us since the 1980s, now embarking on their retirements, and welcome a new generation of researchers. -
Kayla Iacovino, Ph.D. Curriculum Vitae
Kayla Iacovino, Ph.D. Curriculum Vitae Arizona State University Phone: +1 (480) 727-2558 School of Earth and Space Exploration Email: [email protected] 781 E Terrace Rd. www.kaylaiacovino.com Tempe, AZ 85287-6004 @kaylai EDUCATION 2010–14 Ph.D, University of Cambridge An unexpected journey: Experimental insights into magma and volatile transport beneath Erebus volcano, Antarctica Supervisor: Dr. Clive Oppenheimer 2010 B.S., Arizona State University, Cum Laude Geological Sciences (minor in Geography) Undergraduate research supervisor: Dr. Gordon Moore PROFESSIONAL EXPERIENCE 2016 – present Post-doctoral Research Scientist, School of Earth and Space Exploration, Arizona State University 2014 – 2016 NSF Post-doctoral Fellow, U.S. Geological Survey, Menlo Park, CA NSF EAR Grant (PI): Quantifying total volatile budgets of explosive volcanic eruptions 2015 – 2016 Visiting Scholar, Dept. of Geological & Environmental Sciences, Stanford University 2010 – 2014 Graduate Researcher, Dept. of Geography, University of Cambridge 2007 – 2010 Undergraduate NASA/Space Grant Research Intern, School of Earth and Space Exploration, Arizona State University 2007 Undergraduate Research Intern, Research Experience for Undergraduates Summer Program, Dept. of Earth Sciences, University of Minnesota PEER REVIEWED PUBLICATIONS h-index: 4 Citations: 36 (via Google Scholar) 8. de Moore M, Aiuppa A, Fischer T, Iacovino K, Stix J, et al (in prep) Sulfur sealing and remobilization during Phreatomagmatic eruptions at Poas observed by gas monitoring using MultiGAS, DOAS, and drones 7. Iacovino K, Till C (submitted) DensityX: A program for calculating the densities of hydrous magmatic liquids from 327-1,727 °C and up to 30 kbar, Volcanica. 6. Lowenstern JB, van Hinsberg V, Berlo K, Liesegang M, Iacovino K, Bindeman I, Wright H (2018) Opal-A in Glassy Pumice, Acid Alteration, and the 1817 Phreatomagmatic Eruption at Kawah Ijen (Java), Indonesia, Frontiers in Volcanology 6:11. -
Kayla Iacovino, Ph.D. Curriculum Vitae
Kayla Iacovino, Ph.D. Curriculum Vitae Jacobs, NASA Johnson Space Center Phone: +1 (281) 792-7884 2101 NASA Pkwy Email: [email protected] Mail Code XI3 www.kaylaiacovino.com Houston, TX 77058 @kaylai EDUCATION 2010–14 Ph.D, University of Cambridge An unexpected journey: Experimental insights into magma and volatile transport beneath Erebus volcano, Antarctica Supervisor: Dr. Clive Oppenheimer 2010 B.S., Arizona State University, Cum Laude Geological Sciences (minor in Geography) Undergraduate research supervisor: Dr. Gordon Moore PROFESSIONAL EXPERIENCE 2019 – Present Research Scientist, Jacobs, NASA Johnson Space Center, Houston, TX 2016 – 2019 Post-doctoral Research Scientist, School of Earth and Space Exploration, Arizona State University 2014 – 2016 NSF Post-doctoral Fellow, U.S. Geological Survey, Menlo Park, CA NSF EAR Grant (PI): Quantifying total volatile budgets of explosive volcanic eruptions 2015 – 2016 Visiting Scholar, Dept. of Geological & Environmental Sciences, Stanford University 2010 – 2014 Graduate Researcher, Dept. of Geography, University of Cambridge 2007 – 2010 Undergraduate NASA/Space Grant Research Intern, School of Earth and Space Exploration, Arizona State University 2007 Undergraduate Research Intern, Research Experience for Undergraduates Summer Program, Dept. of Earth Sciences, University of Minnesota PEER REVIEWED PUBLICATIONS h-index: 5 Citations: 75 (via Google Scholar) 11. Iacovino K, Guild MR, Till CB (in prep) Experimentally determined fO2 of antigorite breakdown fluids and oxidation of the sub-arc mantle 10. Edmonds M, Tutolo B, Iacovino K, Moussallam Y (submitted) Magmatic carbon outgassing and uptake of CO2 by alkaline waters, American Mineralogist. 9. Ojha L, Karunatillake S, Iacovino K (accepted) Atmospheric injection of sulfur from the Medusae Fossae forming events, Planetary and Space Science. -
The Antarctic Sun, January 14, 2007
January 14, 2007 By Peter Rejcek Sun staff MOUNT EREBUS is famous for its per- sistent but low-level activity as the world’s southernmost active volcano. But last year it threw one of its biggest recorded tantrums during its last 165 years. For the second half of 2005, Erebus erupt- ed as much as six times a day, throwing what volcanologists call “bombs,” hot rocks, out of the crater and onto the sides of the 3,794- meter-high volcano. See EREBUS on page 7 Clive Oppenheimer / Special to The Antarctic Sun Quote of Reaching Out ANDRILL completes the Week fi rst core recovery Scientists go online “They should By Peter Rejcek ANDRILL co-chief sci- put a story to educate public Sun staff entist Ross Powell a day The field season may after the drilling opera- about us in By Steve Martaindale be over for the ANtarctic tion ended on Dec. 26. the paper.” Sun staff geological DRILLing The long mosaic The Web site of veteran Antarctic research- program, but the work is of glacial rock types, – Burger bar cook er Sam Bowser sums it up well: only really beginning for diatomite, volcanic impressed with the “Science is useless unless it’s shared, and the scores of ANDRILL ash, siltstone and mud- speed at which his most kids are born scientists. (What do you scientists who will study stone will tell scientists crew turned out grub expect from a species that’s asking ‘Why?’ the sediment core that much about the vari- on a recent night. by the time they turn 3?) We believe in get- was extracted from under ability of Antarctica’s ting people involved with science, no matter the Ross Ice Shelf over paleoclimate and how Inside their age or experience level” (bowserlab. -
Eruptions That Shook the World Clive Oppenheimer Frontmatter More Information
Cambridge University Press 978-0-521-64112-8 - Eruptions that Shook the World Clive Oppenheimer Frontmatter More information Eruptions that Shook the World In April 2010 Eyjafjallajçkull volcano on Iceland belched out an ash cloud that shut down much of Europes airspace for nearly a week. Although only a relatively small eruption, this precipitated the highest level of air travel disruption since the Second World War and it is estimated to have cost the airline industry worldwide over two billion US dollars. But what does it take for a volcanic eruption to really shake the world? Did volcanic eruptions extinguish the dinosaurs? Did they help humans to evolve and conquer the world, only to decimate their populations with a super-eruption 73,000 years ago? Did they contribute to the ebb and flow of ancient empires, the French Revolution, and the rise of fascism in Europe in the nineteenth century? These are some of the claims made for volcanic cataclysm. In this book, volcanologist Clive Oppenheimer explores rich geological, historical, archaeological and paleoenvironmental records (such as ice cores and tree rings) to tell the stories behind some of the greatest volcanic events of the past quarter of a billion years. He shows how a forensic approach to volcanology reveals the richness and complexity behind cause and effect, and argues that important lessons for future catastrophe risk management can be drawn from understanding events that took place even at the dawn of human origins. C L I V E O P P E N H E I M E R is a Reader in Volcanology and Remote Sensing at the University of Cambridge, and a Research Associate of Le Studium Institute for Advanced Studies at ISTO (University of Orle·ans/CNRS). -
Erebus Volcano: in the Footsteps of Shackleton and Scott
H-Announce Erebus Volcano: In the Footsteps of Shackleton and Scott Announcement published by Lucy Dale on Monday, October 29, 2018 Type: Lecture Date: November 18, 2018 Location: United Kingdom Subject Fields: Environmental History / Studies, Geography, History of Science, Medicine, and Technology Location: The National Maritime Museum, Greenwich, London, SE10 9NF Date andTime: 18 November, 11.30 am - 12.30 pm Clive Oppenheimer explains what has been learned about how volcanoes work from observations of the southernmost active volcano, Mount Erebus on Ross Island, Antarctica. The volcanic island was first sighted in 1841 by members of James Clark Ross’ expedition aboard HMSErebus and HMS Terror. Six decades later, it was the base for missions led by Shackleton and Scott. Their pioneering studies paved the way for systematic geological investigations that got underway a further six decades on, and the physical traces they left behind on Ross Island provide a tangible link between past and present scientific endeavours. Clive Oppenheimer is Professor of Volcanology at the University of Cambridge, a writer and filmmaker. He researches volcanic processes and hazards and has a penchant for volcanic degassing and fieldwork in remote places. He spent 13 field seasons investigating Mount Erebus, Antarctica with the US Antarctic Program. His book on large eruptions of the past,Eruptions that Shook the World, inspired the feature film, Into the Inferno that he made with Werner Herzog. All sessions are free but space is limited so to avoid disappointment you will need to book your seat prior to coming to the Museum. https://www.eventbrite.co.uk/e/erebus-volcano-in-the-footsteps-of-shackl.. -
Scott Polar Research Institute Review 2012
Scott Polar Research Institute Review 2012 86th Annual Report of the Scott Polar Research Institute University of Cambridge, UK Captain Scott Memorial in Waterloo Place, London Contents Director’s Introduction .......................................................................................2 Institute Staff ....................................................................................................4 Polar Research ..................................................................................................6 Research Group Structure Polar Physical Science Polar Social Science and Humanities Current Research Grants Publications by Institute Staff ..........................................................................14 Books Papers in Peer-Reviewed Journals Chapters in Books and Other Contributions Doctoral and Masters Theses Seminars Polar Information and Historic Archives ...........................................................17 Library and Information Service World Data Centre for Glaciology, Cambridge Picture Library Archives Polar Record SPRI Website Teaching, Learning and Understanding ...........................................................20 University Teaching The Polar Museum Education and Outreach Projecting the Significance of the Polar Regions Expedition Support: Gino Watkins Fund External Contributions to Polar Activities .........................................................23 National and International Roles of Staff International Glaciological Society (IGS) Scientific Committee on Antarctic -
Volcanic and Magmatic Related Phd Studentships - 2017
Volcanic and Magmatic Related PhD Studentships - 2017 Contents UK NERC Doctoral Training Partnerships The Oxford DTP in Environmental Research Adapting to the Challenges of a Changing SCENARIO: Postgraduate centre in the SCience of Environment (ACCE) the Environment: Natural and Anthropogenic pRocesses, Impacts and Opportunities The Cambridge Earth System Science DTP: Multi- disciplinary studies of the solid Earth, its Science and Solutions for a Changing Planet atmosphere, oceans, cryosphere and biosphere SPITFIRE – the Southampton Partnership for Centre for Doctoral Training in Oil and Gas Innovative Training of Future Investigators Researching the Environment Central England NERC Training Alliance (CENTA) E3 - Edinburgh, Earth and Environment Other Universities not part of a Doctoral Environment East (EnvEast) Training Partnership ENVISION Keele University The Great Western Four Plus (GW4+) LMU, Munich, Germany IAPETUS: Postgraduate Environmental Training Trinity College, Dublin NexUS University College, Dublin The London NERC Doctoral Training Partnership University of Hull The Manchester and Liverpool Doctoral Training Programme University of Iceland NERC Science @ Leeds and York – Site for PhD University of Portsmouth Training in Environmental Research (SPHERES) Uppsala University, Sweden UK NERC Doctoral Training Partnerships Adapting to the Challenges of a Changing Environment (ACCE) University of Sheffield, University of York, Liverpool & other partners http://acce.group.shef.ac.uk/ Host institution: University of Sheffield N/A