Substitution Rates at Neutral Genes Depend on Population Size Under Fluctuating Demography and Overlapping Generations
Total Page:16
File Type:pdf, Size:1020Kb
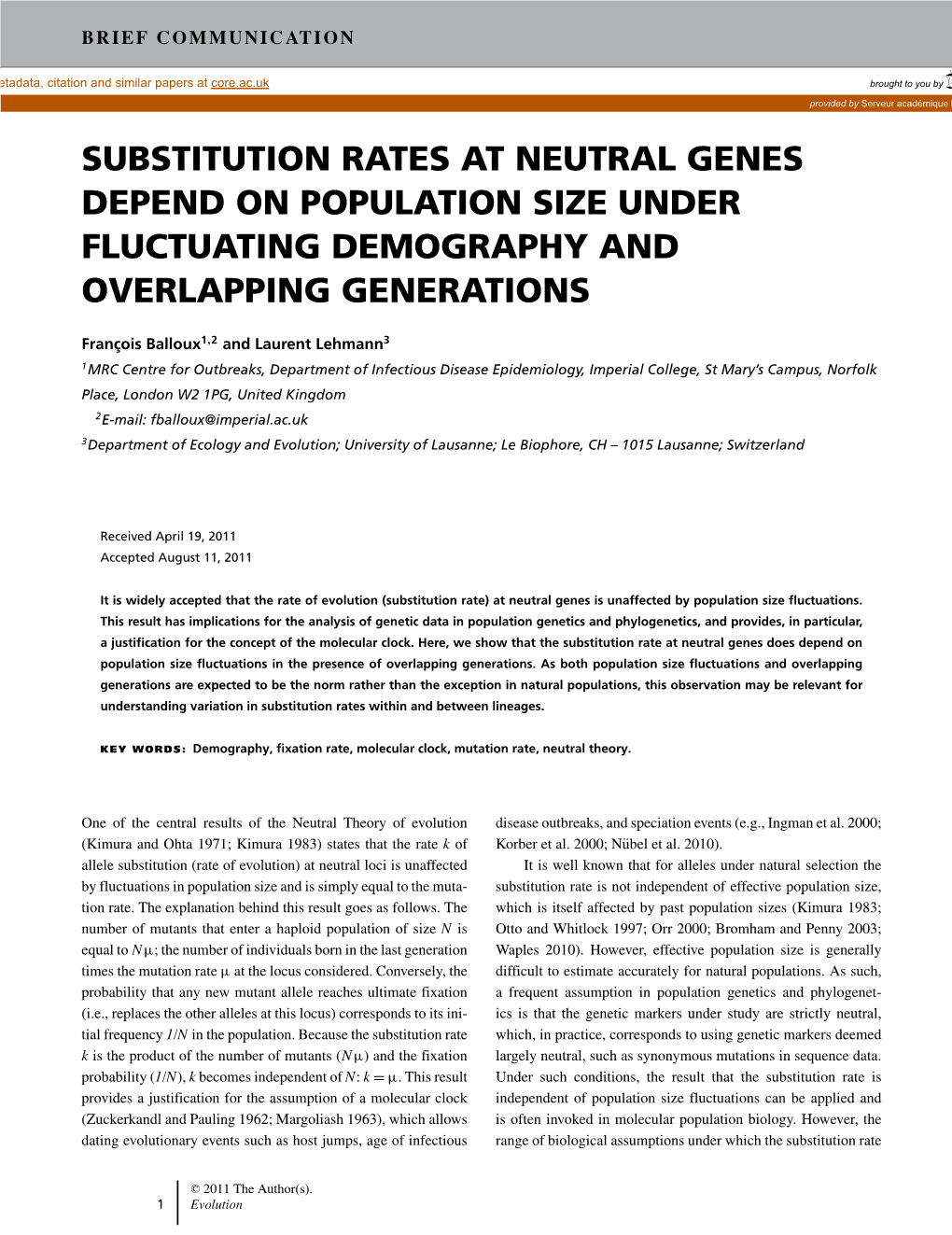
Load more
Recommended publications
-
Effective Population Size and Genetic Conservation Criteria for Bull Trout
North American Journal of Fisheries Management 21:756±764, 2001 q Copyright by the American Fisheries Society 2001 Effective Population Size and Genetic Conservation Criteria for Bull Trout B. E. RIEMAN* U.S. Department of Agriculture Forest Service, Rocky Mountain Research Station, 316 East Myrtle, Boise, Idaho 83702, USA F. W. A LLENDORF Division of Biological Sciences, University of Montana, Missoula, Montana 59812, USA Abstract.ÐEffective population size (Ne) is an important concept in the management of threatened species like bull trout Salvelinus con¯uentus. General guidelines suggest that effective population sizes of 50 or 500 are essential to minimize inbreeding effects or maintain adaptive genetic variation, respectively. Although Ne strongly depends on census population size, it also depends on demographic and life history characteristics that complicate any estimates. This is an especially dif®cult problem for species like bull trout, which have overlapping generations; biologists may monitor annual population number but lack more detailed information on demographic population structure or life history. We used a generalized, age-structured simulation model to relate Ne to adult numbers under a range of life histories and other conditions characteristic of bull trout populations. Effective population size varied strongly with the effects of the demographic and environmental variation included in our simulations. Our most realistic estimates of Ne were between about 0.5 and 1.0 times the mean number of adults spawning annually. We conclude that cautious long-term management goals for bull trout populations should include an average of at least 1,000 adults spawning each year. Where local populations are too small, managers should seek to conserve a collection of interconnected populations that is at least large enough in total to meet this minimum. -
Effects of Overlapping Generations on Linkage Disequilibrium Estimates of Effective Population Size
INVESTIGATION Effects of Overlapping Generations on Linkage Disequilibrium Estimates of Effective Population Size Robin S. Waples,*,1 Tiago Antao,† and Gordon Luikart‡ *Northwest Fisheries Science Center, National Marine Fisheries Service, National Oceanic and Atmospheric Administration, Seattle, Washington 98112, †Vector Biology Department, Liverpool School of Tropical Medicine, Liverpool, L3 5QA United Kingdom, and ‡Flathead Lake Biological Station, Fish and Wildlife Genomics Group, Division of Biological Sciences, University of Montana, Polson, Montana 59860 ABSTRACT Use of single-sample genetic methods to estimate effective population size has skyrocketed in recent years. Although the underlying models assume discrete generations, they are widely applied to age-structured species. We simulated genetic data for 21 iteroparous animal and plant species to evaluate two untested hypotheses regarding performance of the single-sample method based on linkage disequilibrium (LD): (1) estimates based on single-cohort samples reflect the effective number of breeders in one reproductive cycle (Nb), and (2) mixed-age samples reflect the effective size per generation (Ne). We calculated true Ne and Nb, using the model species’ vital rates, and verified these with individual-based simulations. We show that single-cohort samples should be ^ 2 equally influenced by Nb and Ne and confirm this with simulated results: Nb was a linear (r = 0.98) function of the harmonic mean of ^ Ne and Nb. We provide a quantitative bias correction for raw Nb based on the ratio Nb/Ne, which can be estimated from two or three simple life history traits. Bias-adjusted estimates were within 5% of true Nb for all 21 study species and proved robust when challenged with new data. -
Effects of Overlapping Generations on Linkage Disequilibrium Estimates of Effective Population Size
INVESTIGATION Effects of Overlapping Generations on Linkage Disequilibrium Estimates of Effective Population Size Robin S. Waples,*,1 Tiago Antao,† and Gordon Luikart‡ *Northwest Fisheries Science Center, National Marine Fisheries Service, National Oceanic and Atmospheric Administration, Seattle, Washington 98112, †Vector Biology Department, Liverpool School of Tropical Medicine, Liverpool, L3 5QA United Kingdom, and ‡Flathead Lake Biological Station, Fish and Wildlife Genomics Group, Division of Biological Sciences, University of Montana, Polson, Montana 59860 ABSTRACT Use of single-sample genetic methods to estimate effective population size has skyrocketed in recent years. Although the underlying models assume discrete generations, they are widely applied to age-structured species. We simulated genetic data for 21 iteroparous animal and plant species to evaluate two untested hypotheses regarding performance of the single-sample method based on linkage disequilibrium (LD): (1) estimates based on single-cohort samples reflect the effective number of breeders in one reproductive cycle (Nb), and (2) mixed-age samples reflect the effective size per generation (Ne). We calculated true Ne and Nb, using the model species’ vital rates, and verified these with individual-based simulations. We show that single-cohort samples should be ^ 2 equally influenced by Nb and Ne and confirm this with simulated results: Nb was a linear (r = 0.98) function of the harmonic mean of ^ Ne and Nb. We provide a quantitative bias correction for raw Nb based on the ratio Nb/Ne, which can be estimated from two or three simple life history traits. Bias-adjusted estimates were within 5% of true Nb for all 21 study species and proved robust when challenged with new data. -
Temporal Estimates of Effective Population Size in Species with Overlapping Generations
View metadata, citation and similar papers at core.ac.uk brought to you by CORE provided by DigitalCommons@University of Nebraska University of Nebraska - Lincoln DigitalCommons@University of Nebraska - Lincoln Publications, Agencies and Staff of the U.S. Department of Commerce U.S. Department of Commerce 2007 Temporal Estimates of Effective Population Size in Species With Overlapping Generations Robin Waples NOAA, [email protected] Masashi Yokota Northwest Fisheries Science Center Follow this and additional works at: https://digitalcommons.unl.edu/usdeptcommercepub Waples, Robin and Yokota, Masashi, "Temporal Estimates of Effective Population Size in Species With Overlapping Generations" (2007). Publications, Agencies and Staff of the U.S. Department of Commerce. 458. https://digitalcommons.unl.edu/usdeptcommercepub/458 This Article is brought to you for free and open access by the U.S. Department of Commerce at DigitalCommons@University of Nebraska - Lincoln. It has been accepted for inclusion in Publications, Agencies and Staff of the U.S. Department of Commerce by an authorized administrator of DigitalCommons@University of Nebraska - Lincoln. Copyright Ó 2007 by the Genetics Society of America DOI: 10.1534/genetics.106.065300 Temporal Estimates of Effective Population Size in Species With Overlapping Generations Robin S. Waples*,1 and Masashi Yokota*,† *Northwest Fisheries Science Center, Seattle, Washington 98112 and †Tokyo University of Marine Science and Technology, Tokyo 108-8477, Japan Manuscript received August 25, 2006 Accepted for publication October 18, 2006 ABSTRACT The standard temporal method for estimating effective population size (Ne) assumes that generations are discrete, but it is routinely applied to species with overlapping generations. We evaluated bias in the estimates Nˆe caused by violation of this assumption, using simulated data for three model species: humans (type I survival), sparrow (type II), and barnacle (type III). -
Estimation of Census and Effective Population Sizes: the Increasing Usefulness of DNA-Based Approaches
Conserv Genet (2010) 11:355–373 DOI 10.1007/s10592-010-0050-7 REVIEW Estimation of census and effective population sizes: the increasing usefulness of DNA-based approaches Gordon Luikart • Nils Ryman • David A. Tallmon • Michael K. Schwartz • Fred W. Allendorf Received: 16 October 2009 / Accepted: 11 January 2010 / Published online: 27 February 2010 Ó Springer Science+Business Media B.V. 2010 Abstract Population census size (NC) and effective estimators of both NC and Ne, and the often undervalued population sizes (Ne) are two crucial parameters that and misunderstood ratio of effective-to-census size influence population viability, wildlife management deci- (Ne/NC). We focus on recently improved and well evalu- sions, and conservation planning. Genetic estimators of ated methods that are most likely to facilitate conservation. both NC and Ne are increasingly widely used because Finally, we outline areas of future research to improve Ne molecular markers are increasingly available, statistical and NC estimation in wild populations. methods are improving rapidly, and genetic estimators complement or improve upon traditional demographic Keywords Population size estimation Á estimators. We review the kinds and applications of Noninvasive sampling Á Remote genetic monitoring Á Abundance Á Bottleneck Á Ne/NC ratio Á Habitat fragmentation G. Luikart (&) Á F. W. Allendorf Division of Biological Sciences, University of Montana, Missoula, MT 59812, USA e-mail: [email protected] Counting fish is like counting trees, except they are G. Luikart invisible and they keep moving. Centro de Investigac¸a˜o em Biodiversidade e Recursos Gene´ticos and Universidade do, Porto (CIBIO-UP), 4485-661 Vaira˜o, John Shepherd (from Hilborn 2002) Portugal Effective population size (Ne) is a critical parameter Present Address: in population biology, but it is difficult to collect G. -
Effect of Harvest and Effective Population Size on Genetic Diversity in a Striped Bass Population
University of South Carolina Scholar Commons Faculty Publications Biological Sciences, Department of 11-2000 Effect of Harvest and Effective Population Size on Genetic Diversity in a Striped Bass Population Marilyn Diaz University of South Carolina - Columbia David S. Wethey University of South Carolina - Columbia, [email protected] James Bulak University of South Carolina - Columbia Bert Ely University of South Carolina - Columbia, [email protected] Follow this and additional works at: https://scholarcommons.sc.edu/biol_facpub Part of the Biology Commons Publication Info Transactions of the American Fisheries Society, Volume 129, Issue 6, 2000, pages 1367-1372. © Transactions of the American Fisheries Society 2000, American Fisheries Society. This Article is brought to you by the Biological Sciences, Department of at Scholar Commons. It has been accepted for inclusion in Faculty Publications by an authorized administrator of Scholar Commons. For more information, please contact [email protected]. Transactions of the American Fisheries Society 129:1367±1372, 2000 q Copyright by the American Fisheries Society 2000 Effect of Harvest and Effective Population Size on Genetic Diversity in a Striped Bass Population MARILYN DIAZ1 AND DAVID WETHEY Department of Biological Sciences, University of South Carolina, Columbia, South Carolina 29208, USA JAMES BULAK Department of Biological Sciences, University of South Carolina, Columbia, South Carolina 29208, USA and South Carolina Department of Natural Resources, Eastover, South Carolina 29044, USA BERT ELY* Department of Biological Sciences, University of South Carolina, Columbia, South Carolina 29208, USA Abstract.ÐA major factor that contributes to loss of population size, particularly if sustained over sev- genetic variation in natural populations is a small ef- eral generations (Wright 1938; Nei et al. -
Analyzing the Neutral and Adaptive Background of Butterfly Voltinism Reveals Structural Variation in a Core Circadian Gene
bioRxiv preprint doi: https://doi.org/10.1101/2020.05.13.093310; this version posted May 15, 2020. The copyright holder for this preprint (which was not certified by peer review) is the author/funder, who has granted bioRxiv a license to display the preprint in perpetuity. It is made available under aCC-BY-NC-ND 4.0 International license. Lindestad et al. Pre-print manuscript, May 2020 Analyzing the neutral and adaptive background of butterfly voltinism reveals structural variation in a core circadian gene Olle Lindestad1, Sören Nylin, Christopher W. Wheat2 & Karl Gotthard2 1 Corresponding author 2 These authors contributed equally to this work Department of Zoology, Stockholm University ABSTRACT Many insects exhibit geographic variation in voltinism, the number of generations produced per year. This includes high-latitude species in previously glaciated areas, implying divergent selection on life cycle traits during or shortly after recent colonization. Here, we use a whole- genome approach to genetically characterize a set of populations of the butterfly Pararge aegeria that differ in voltinism. We construct a high-quality de novo genome for P. aegeria, and assess genome-wide genetic diversity and differentiation between populations. We then use the inferred phylogeographic relationships as the basis for a scan for loci showing signs of divergent selection associated with voltinism differences. The genic outliers detected include population-specific mutations of circadian loci, most notably a locally fixed 97-amino acid de- letion in the circadian gene timeless. Variation in timeless has previously been implicated as underlying variation in life cycle regulation in wild populations in our study species, as well as in other insects. -
Prediction and Estimation of Effective Population Size
Heredity (2016) 117, 193–206 & 2016 Macmillan Publishers Limited, part of Springer Nature. All rights reserved 0018-067X/16 www.nature.com/hdy REVIEW Prediction and estimation of effective population size J Wang1, E Santiago2 and A Caballero3 Effective population size (Ne) is a key parameter in population genetics. It has important applications in evolutionary biology, conservation genetics and plant and animal breeding, because it measures the rates of genetic drift and inbreeding and affects the efficacy of systematic evolutionary forces, such as mutation, selection and migration. We review the developments in predictive equations and estimation methodologies of effective size. In the prediction part, we focus on the equations for populations with different modes of reproduction, for populations under selection for unlinked or linked loci and for the specific applications to conservation genetics. In the estimation part, we focus on methods developed for estimating the current or recent effective size from molecular marker or sequence data. We discuss some underdeveloped areas in predicting and estimating Ne for future research. Heredity (2016) 117, 193–206; doi:10.1038/hdy.2016.43; published online 29 June 2016 INTRODUCTION which correspond to the so-called inbreeding and variance effective The concept of effective population size, introduced by Sewall Wright sizes, respectively (Crow and Kimura, 1970). (1931, 1933), is central to plant and animal breeding (Falconer and Predictions of the effective population size can also be obtained Mackay, 1996), conservation genetics (Frankham et al.,2010; from the largest nonunit eigenvalue of the transition matrix of a Allendorf et al., 2013) and molecular variation and evolution Markov Chain which describes the dynamics of allele frequencies. -
Developments in the Prediction of Effective Population Size
Heredity73 (1994) 657—679 Received9 March 1994 Genetical Society of Great Britain Review article Developments in the prediction of effective population size ARMANDO CABALLERO Institute of Cell, Animal and Population Biology, University of Edinburgh, West Mains Road, Edinburgh EH9 3JT, UK Effectivepopulation size is a key parameter in evolutionary and quantitative genetics because it measures the rate of genetic drift and inbreeding. Predictive equations of effective size under a range of circumstances and some of their implications are reviewed in this paper. Derivations are made for the simplest cases, and the inter-relations between different formulae and methods are discussed. Keywords: heterozygosity, inbreeding, nonrandom mating, population numbers, random genetic drift. Contents 1 The idealized population and effective population size 2 Self-fertilization not allowed 3 Different numbers of male and female parents 4 Variable population size over generations 5 Nonrandom contribution from parents 5.1 Variation due to noninhented causes 5.1.1 Monoecious diploid species with selfing allowed 5.1.2 Separate sexes 5.1.3 Haploid and polyploid species 5.1.4 X-linked genes 5.1.5 Systems of mating 5.2 Variation due to inherited causes 5.2.1 Effective size from the variance of change in gene frequency over generations 5.2.2 Selection on fitness 5.2.3 Effective size from long-term contributions of ancestors 5.2.4 Index selection 6 Nonrandom mating with population subdivision 7 Overlapping generations 8 Prospects for future developments References 1 Theidealized populationand effective thefinite sampling of gametes. These erratic changes populationsize constitutethe so-called dispersive process or genetic In an infinitely large population and in the absence of drift, which is likely to be one of the main factors mutation, migration and selection, gene and genotype governing molecular evolution (Kimura, 1983) and has frequencies remain constant over generations. -
Role of Overlapping Generations in Maintaining Genetic Variation in a Fluctuating Environment
Vol. 143, No. 3 The American Naturalist March 1994 ROLE OF OVERLAPPING GENERATIONS IN MAINTAINING GENETIC VARIATION IN A FLUCTUATING ENVIRONMENT STEPHEN ELLNER* AND NELSON G. HAIRSTON, JR.tt *Biomathematics Program, Department of Statistics, North Carolina State University, Raleigh, North Carolina 27695-8203; tSection of Ecology and Systematics, Cornell University, Ithaca, New York 14853-2701 Submitted September 17, 1992; Revised April 19, 1993; Accepted May 19, 1993 Abstract.-Population genetics theory suggests that temporally fluctuating selection on pheno- types can act to maintain genetic variance only under very restrictive conditions. However, this conclusion is based on models with discrete nonoverlapping generations. We propose here that temporally fluctuating selection can indeed contribute significantly to the maintenance of genetic variation when the effects of overlapping generations and age-specific or stage-specific selection are considered. We develop a simple model for a population with overlapping generations, experiencing stabilizing selection with a temporally fluctuating optimum, and subject to repeated invasions by mutants with alternative phenotypes. We find that an evolutionarily stable popula- tion must have positive genetic variance maintained by selection so long as the product (variance of fluctuations) times (amount of generation overlap) times (selection intensity) is sufficiently high. This result applies to haploid, diploid, single-locus, or multilocus inheritance, and it does not depend on any form of heterozygote advantage to maintain genetic variance. However, it depends on the map between genotype and phenotype being constrained. If a single genotype can produce an arbitrary distribution of phenotypes, then genetic variance is not maintained by selection. Temporally fluctuating selection, in which the relative fitness of different phe- notypes varies over time, has repeatedly been proposed and rejected as a signifi- cant process maintaining genetic variation in natural populations (Hedrick et al. -
Temporal Estimates of Effective Population Size in Species with Overlapping Generations
Copyright Ó 2007 by the Genetics Society of America DOI: 10.1534/genetics.106.065300 Temporal Estimates of Effective Population Size in Species With Overlapping Generations Robin S. Waples*,1 and Masashi Yokota*,† *Northwest Fisheries Science Center, Seattle, Washington 98112 and †Tokyo University of Marine Science and Technology, Tokyo 108-8477, Japan Manuscript received August 25, 2006 Accepted for publication October 18, 2006 ABSTRACT The standard temporal method for estimating effective population size (Ne) assumes that generations are discrete, but it is routinely applied to species with overlapping generations. We evaluated bias in the estimates Nˆe caused by violation of this assumption, using simulated data for three model species: humans (type I survival), sparrow (type II), and barnacle (type III). We verify a previous proposal by Felsenstein that weighting individuals by reproductive value is the correct way to calculate parametric population allele frequencies, in which case the rate of change in age-structured populations conforms to that predicted by discrete-generation models. When the standard temporal method is applied to age- structured species, typical sampling regimes (sampling only newborns or adults; randomly sampling the entire population) do not yield properly weighted allele frequencies and result in biased Nˆe. The direction and magnitude of the bias are shown to depend on the sampling method and the species’ life history. Results for populations that grow (or decline) at a constant rate paralleled those for populations of constant size. If sufficient demographic data are available and certain sampling restrictions are met, the Jorde–Ryman modification of the temporal method can be applied to any species with overlapping generations.