El Niño and It's Currents
Total Page:16
File Type:pdf, Size:1020Kb
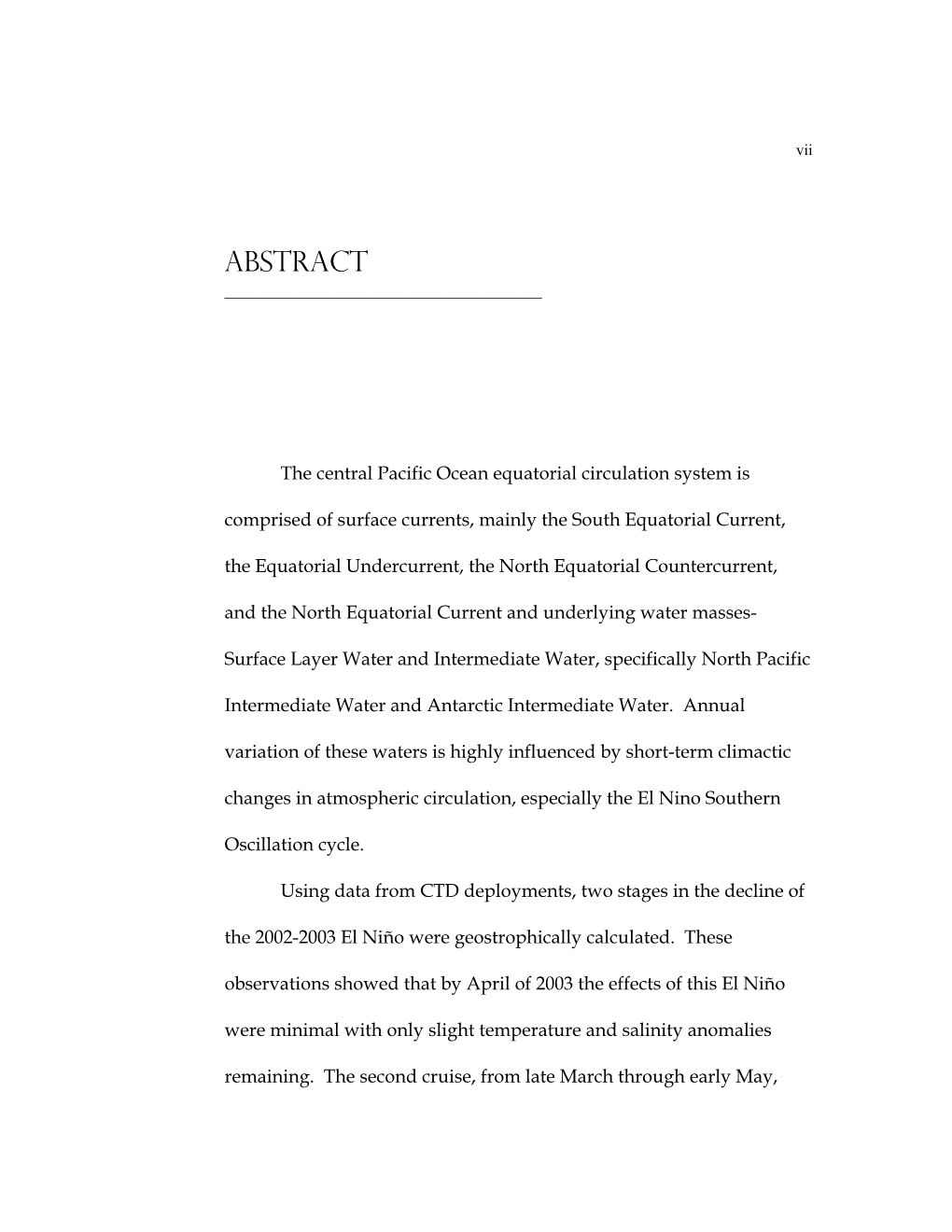
Load more
Recommended publications
-
Tropical Meteorology
Tropical Meteorology Chapter 01B Topics ¾ El Niño – Southern Oscillation (ENSO) ¾ The Madden-Julian Oscillation ¾ Westerly wind bursts 1 The Southern Oscillation ¾ There is considerable interannual variability in the scale and intensity of the Walker Circulation, which is manifest in the so-called Southern Oscillation (SO). ¾ The SO is associated with fluctuations in sea level pressure in the tropics, monsoon rainfall, and wintertime circulation over the Pacific Ocean and also over North America and other parts of the extratropics. ¾ It is the single most prominent signal in year-to-year climate variability in the atmosphere. ¾ It was first described in a series of papers in the 1920s by Sir Gilbert Walker and a review and references are contained in a paper by Julian and Chervin (1978). The Southern Oscillation ¾ Julian and Chervin (1978) use Walker´s own words to summarize the phenomenon. "By the southern oscillation is implied the tendency of (surface) pressure at stations in the Pacific (San Francisco, Tokyo, Honolulu, Samoa and South America), and of rainfall in India and Java... to increase, while pressure in the region of the Indian Ocean (Cairo, N.W. India, Darwin, Mauritius, S.E. Australia and the Cape) decreases...“ and "We can perhaps best sum up the situation by saying that there is a swaying of pressure on a big scale backwards and forwards between the Pacific and Indian Oceans...". 2 Fig 1.17 The Southern Oscillation ¾ Bjerknes (1969) first pointed to an association between the SO and the Walker Circulation, but the seeds for this association were present in the studies by Troup (1965). -
Examining the Effect of El Nino Phenomena and Pacific Sea Surface Temperature on the Climate of the Glacierized White Mountains in Peru
Bridgewater State University Virtual Commons - Bridgewater State University Honors Program Theses and Projects Undergraduate Honors Program 5-12-2020 Examining the Effect of El Nino Phenomena and Pacific Sea Surface Temperature on the Climate of the Glacierized White Mountains in Peru Emily Reardon Bridgewater State University Follow this and additional works at: https://vc.bridgew.edu/honors_proj Part of the Physics Commons Recommended Citation Reardon, Emily. (2020). Examining the Effect of El Nino Phenomena and Pacific Sea Surface Temperature on the Climate of the Glacierized White Mountains in Peru. In BSU Honors Program Theses and Projects. Item 349. Available at: https://vc.bridgew.edu/honors_proj/349 Copyright © 2020 Emily Reardon This item is available as part of Virtual Commons, the open-access institutional repository of Bridgewater State University, Bridgewater, Massachusetts. Examining the Effect of El Nino Phenomena and Pacific Sea Surface Temperature on the Climate of the Glacierized White Mountains in Peru Emily Reardon Submitted in Partial Completion of the Requirements for Departmental Honors in Physics Bridgewater State University May 12, 2020 Dr. Robert Hellstrom, Thesis Advisor Dr. Thomas Kling, Committee Member Dr. Jeffrey Williams, Committee Member Examining the Effect of El Nino Phenomena and Pacific Sea Surface Temperature on the Climate of the Glacierized White Mountains in Peru Emily Reardon, Mentor Dr. Hellstr¨om Physics Department Bridgewater State University, MA May 12, 2020 Abstract The purpose of this study is to determine if there is a correlation between the El Ni~noSouthern Oscillation, sea surface temperatures (SST) and the climate of the Rio Santa Basin. This study is an important step in understanding the dynamics of the glaciers as a critical control on hydrological features in alpine Andes Valleys. -
Climatological Seesaws in the Southwest Pacific
Weather and Climate (1993) 13: 9-21 9 CLIMATOLOGICAL SEESAWS IN THE SOUTHWEST PACIFIC John Hayl Jim Salinger2 Blair Fitzharris3 and Reid Basher2 1 Environmental Science, University of Auckland, Auckland, New Zealand Postal Address: Environmental Science, University of Auckland, Private Bag 92019, Auckland, New Zealand Telephone: 649-373-7599 (Extn 8437) Facsimile: 649-373-7470 2 National Institute of Water and Atmospheric Research, Wellington, New Zealand 3 Geography Department, University of Otago, Dunedin, New Zealand ABSTRACT The analyses reported in this paper are based on an unprecedented 80 year record of reconstructed grid-point sea-level pressure data which are used to derive composite pressure anomaly fields for the Southwest Pacific for extreme phases of the Southern Oscillation. These show centres of action to the east and west of New Zealand. However, it is the intervening pivotal region that includes New Zealand which experiences a strong response — anomalous south to southwest flow when the Southern Oscillation Index (SOI) is negative and north to northeasterly flow when the Index is positive. These contrasting responses are reflected in the spatial distribution of rainfall anomalies for New Zealand. A more complex response occurs in another pivotal region in the vicinity of the Southern Cook Islands. The South Pacific Convergence Zone is displaced northward and southward in association with extreme phases of the Southern Oscillation resulting in large rainfall anomalies in the areas which are under its influence only in such conditions. However, the effective finite width of the South Pacific Convergence Zone and its limited north/south displacement mean that there are some locations in the pivotal region which are always influenced by the convergence zone. -
Constructing the Monsoon: Colonial Meteorological Cartography, 1844– 1944
Constructing the Monsoon: Colonial Meteorological Cartography, 1844– 1944 Beth Cullen & Christina Leigh Geros Introduction During the nineteenth century, the significance of the South Asian monsoon for the expanding the British Empire made it one of the most anticipated, tracked, and studied weather phenomena in the world.1 Innovations in mapping techniques and scientific instruments, as well as advancements in communication technology, converged with the establishment of colonial rule in South Asia, bringing the monsoon into focus.2 This paper explores developments in meteorological cartography that highlight constructions of the monsoon during the nineteenth and early twentieth centuries. The early nineteenth century saw a shift in meteorological inquiry towards standardisation, quantification, and synchronisation, which led to the rise of new representational techniques, namely statistical cartography.3 Weather maps were one of the most remarkable innovations of nineteenth-century meteorology, their emergence an attempt to better understand the invisible forces of the atmosphere.4 The use of statistical data to create meteorological images made it possible to identify global atmospheric patterns for the first time. Through these new strategies of visualisation, weather patterns were given shape and form, graphically and geographically, thereby enabling weather phenomena to become the object of scientific inquiry.5 Nineteenth-century statistical cartographies, based on systematic data collection, were a significant advance in scientific knowledge production and played an important role in discovering new meteorological objects of inquiry. The art of 1 Sunil Amrith, “Risk and the South Asian Monsoon,” Climatic Change 151, no. 17 (2016): 17-28; Mike Davis, Late Victorian Holocausts: El Nino Famines and the Making of the Third World (London and New York: Verso, 2002). -
Rothamsted in the Making of Sir Ronald Fisher Sc.D., F.R.S
Rothamsted in the Making of Sir Ronald Fisher Sc.D., F.R.S. John Aldrich Economics Department University of Southampton Southampton SO17 1BJ UK e-mail: [email protected] Abstract In 1919 the agricultural station at Rothamsted hired Ronald Fisher (1890-1962) to analyse historic data on crop yields. For him it was the beginning of a spectacular career and for Rothamsted the beginning of a Statistics Department which became a force in world statistics. Fisher arrived with publications in mathematical statistics and genetics. These were established subjects but at Rothamsted between 1919 and -33 he created the new career of agricultural research statistician. The paper considers how Rothamsted, in the person of its Director Sir John Russell, nurtured this development while supporting Fisher’s continuing work in mathematical statistics and genetics. It considers too how people associated with Fisher at Rothamsted, including assistants Wishart, Irwin and Yates and voluntary workers Tippett, Hoblyn and Hotelling contributed to establishing Fisherian statistics. September 2019 1 Introduction In 1919 a 29 year-old Cambridge mathematics graduate with no established profession started at Rothamsted Experimental Station; he died in 1962 “the most famous statistician and mathematical biologist in the world”—thus Irwin et al. (1963: 159). Sir Ronald Fisher Sc.D., F.R.S. was Balfour Professor at Cambridge when he was knighted and had been Galton Professor at University College but the higher doctorate and Fellowship of the Royal Society came when he was at Rothamsted. Ronald Aylmer Fisher would be another Galton and Balfour Professor but at Rothamsted he was the first Chief Statistician or even statistician. -
Impact of El Niño Southern Oscillation on Monsoon Rainfall in Bhima Basin, Central India
City University of New York (CUNY) CUNY Academic Works International Conference on Hydroinformatics 2014 Impact Of El Niño Southern Oscillation On Monsoon Rainfall In Bhima Basin, Central India Dhyan Singh Arya Mohamed Gareeballa Abdall Asmita Ramkrishna Murumkar How does access to this work benefit ou?y Let us know! More information about this work at: https://academicworks.cuny.edu/cc_conf_hic/197 Discover additional works at: https://academicworks.cuny.edu This work is made publicly available by the City University of New York (CUNY). Contact: [email protected] 11th International Conference on Hydroinformatics HIC 2014, New York City, USA IMPACT OF EL NIÑO SOUTHERN OSCILLATION ON SUMMER MONSOON RAINFALL IN BHIMA BASIN, CENTRAL INDIA D.S. ARYA (1), A. R. MURUMKAR (1), A. GAREEBALLA (1) (1): Department of Hydrology, IIT Roorkee, Roorkee 247 667, Uttarakhand, India The study of the effects of ENSO on summer monsoon rainfall (SMR) was carried in the Bhima River basin located in Central India. Gridded 10x10 resolution daily rainfall data (1901-2004) and ENSO indices data (SOI, MEI and Niño 3.4) were used in this study. Analysis show that the SMR is significantly correlated with monsoonal ENSO indices at most of the grids. SMR is positively correlated with SOI index whereas negatively correlated with MEI and N3.4 indices. ENSO phase wise analysis shows that a strong/weak SMR is associated with La Niña phase/El Niño phase except for the grids that are located on higher elevation in Western Ghats. Overall on an average 15.02% more rainfall has been received during La Niña phases and 9.50% less in El Niño phases in the basin during summer monsoon season. -
Integrated Global Radiosonde Archive
Detecting and Describing Teleconnections Stefan Liess Department of Soil, Water, and Climate - University of Minnesota [email protected] … General Circulation … (Hadley Phil. Trans. 1735) http://www.meted.ucar.edu/tropical/textbook_2nd_edition/media/graphics/ch3_overview_image.jpg Detecting Climate Change from Data 2 Related Work to find Teleconnections Discovered earlier by human observation. North Atlantic Oscillation observed in 1770-17781 Southern Oscillation observed by Sir Gilbert Walker as a sea-saw like oscillation of sea level pressure in the Pacific Ocean in 19232 EOF analysis used to identify individual Teleconnections for the Northern and Southern Annular Modes (NAM and SAM)3 EOF decomposes the time series into orthogonal basis functions. NAM: EOF Analysis of 20N-90N Latitude (aka Arctic Oscillation) SAM: EOF Analysis of 20S-90S Latitude (aka Antarctic Oscillation) 1. H. van Loon and J. C. Rogers. The seesaw in winter temperatures between Greenland and Northern Europe. Part I: General description. Monthly Weather Review, (1978) 2. G. Walker. Correlation in seasonal variations of weather, viii. a preliminary study of world weather. Memoirs of the India Meteorological Department, (1923) 3. H. Von Storch and F. Zwiers. Statistical analysis in climate research. Cambridge University Press, (2002) 4. Portis, D. H., Walsh, J. E., El Hamly, Mostafa and Lamb, Peter J., Seasonality of the North Atlantic Oscillation, J. Climate, (2001) Motivation for Automatic Discovery of Multiple Teleconnections 4 The known Teleconnections are Dynamic behavior of the defined by static locations but the high and low pressure fields underlying phenomenon is dynamic corresponding to NAO climate index (Portis et al, 2001) Manual discovery can miss many Teleconnections EOF and other types of eigenvector AO: EOF analysis finds the strongest signals Analysis of 20N- and the physical interpretation of 90N Latitude those can be difficult. -
Scanned Using Book Scancenter 5022
Also by Brian Fagan Floods, The Great Warming Fish on Friday Famines, Chaco Canyon The Long Summer and The Little Ice Age From Black Land to Fifth Sun Emperors Eyewitness to Discovery (editor) Oxford Companion to Archaeology (editor) El Nino and the Time Detectives Kingdoms of Jade, Kingdoms of Gold Fate of Civilizations Ancient North America The Journey from Eden The Great Journey The Adventure of Archaeology Revised Edition The Aztecs Clash of Cultures Return to Babylon Elusive Treasure Brian Fagan The Rape of the Nile BASIC BBOOKS A Member of the Perseus Books Group New York Copyright © 1999, 2009 by Brian Fagan Published by Basic Books, A Member of the Perseus Books Group For Lesley All rights reserved. Printed in the United States of America. No part of this book may be reproduced in any manner whatsoever without written When I see you coming my heart beats permission except in the case of brief quotations embodied in critical articles and reviews. For information, address Basic Books, 387 Park Avenue South, New York, NY 10016-8810. harder and I reach for your hand. Books published by Basic Books are available at special discounts for bulk Or if you prefer: light blue touch paper—and stand clear purchases in the United States by corporations, institutions, and other or ganizations. For more information, please contact the Special Markets Department at the Perseus Books Group, 2300 Chestnut Street, Suite 200, Philadelphia, PA 19103, or call (800) 810-4145, ext. 5000, or e-mail [email protected]. Library of Congress Cataloging-in-Publication Data Fagan, Brian M. -
The Zonal Walker Circulation
The Zonal Walker Circulation Introduction In addition to the mean meridional Hadley circulation, there also exist zonal overturning circulations that contribute to the meteorology and climatology of the tropics. Together, these overturning circulations are commonly referred to as the Walker circulation. In this section, we first explore the history and basic structure of the Walker circulation. Later, we reintroduce the shallow-water equations to demonstrate how prescribed heating leads to equatorially trapped Kelvin and equatorial Rossby waves that comprise the Walker circulation. In so doing, we tie these features back to the Hadley cell circulation and provide motivation for subsequent lectures on the El Niño-Southern Oscillation and monsoons. Key Questions • What is the zonal Walker circulation? • What drives the Walker circulation? What is the zonal Walker circulation? As previously discussed, tropical circulations result from heating. More specifically, they result from a combination of both asymmetric and symmetric heating, where symmetry is defined here by the symmetry of the heating source with respect to the Equator. Owing to considerations of continentality and seasonality, the Hadley circulation is an example of an asymmetric heating-driven tropical circulation wherein its rising branch is typically displaced off of the Equator. Conversely, the mean equatorial east- west (or zonal) circulation in the Pacific Ocean is an example of a symmetric heating-driven tropical circulation. This circulation is known as the Walker circulation, named after Sir Gilbert Walker who first recognized its existence from observations of sea-level pressure between Darwin, Australia and the island of Tahiti in the tropical Pacific. In recent years, however, the term “Walker circulation” has come to refer to not just the equatorial zonal circulation in the Pacific but also that of the equatorial zonal circulations across the Atlantic and Indian Oceans as well. -
2 Yule and Hooker and the Concepts of Correlation and Trend
Notes 2 Yule and Hooker and the Concepts of Correlation and Trend 1. Galton is often regarded as the father of correlation, but the concept – or at least the word – had been in common use, particularly in physics, since the middle of the cen- tury. The historical development of correlation, although fascinating, lies outside the scope of this study. A contemporary account of the development is provided by Pear- son (1920): for a later, rather more detached, discussion, see Stigler (1986, chapter 8). 2. George Udny Yule plays a major and recurring role in our story. Born on 18 February 1871 in Beech Hill near Haddington, Scotland, Yule was a member of an established Scottish family composed of army officers, civil servants, scholars and administrators and both his father, also named George Udny, and a nephew were knighted. Although he originally studied engineering and physics at University College, London (UCL), and Bonn, Germany, publishing four papers on electric waves, Yule returned to UCL in 1893, becoming first a demonstrator for Karl Pearson, then an Assistant Professor. Yule left UCL in 1899 to work for the City and Guilds of London Institute but also later held the Newmarch Lectureship in Statistics at UCL. In 1912 he became lecturer in statistics at the University of Cambridge (later being promoted to Reader) and in 1913 began his long association with St. John’s College, becoming a Fellow in 1922. Yule was also very active in the Royal Statistical Society: elected a Fellow in 1895, he served as Honorary Secretary, was President and was awarded the prestigious Guy Medal in Gold in 1911. -
Sir Gilbert Thomas Walker
ARTICLE-I N-A- BOX Sir Gilbert Thomas Walker Sir Gilbert Thomas Walker, a pioneer in the use of statistical correlation, multiple regression techniques and harmonic analysis in weather forecasting, was born on 14 June 1868 at Rochdale, Lancashire. He was educated at St Paul's School and later at the Trinity College, Cambridge. Walker came to India in 1904 to take charge as the Director General of Meteorology at the India Meteorological Department. Though monsoon forecasting in India had begun nearly twenty years earlier in 1886, there was no objective method for forecast ing and rigorous statistical techniques were not adopted till the arrival of Walker. He introduced the concept of correlation and regression for developing objective models based on precursory signals from different parts of the world. In 1909, the first forecast using a regression equation was made. Walker spent the next 21 years doing research on monsoon forecasting. His fundamental premise was that the relations between weather over the earth are so complex that it is seems useless to try and derive them from theoretical considerations. Hence he looked at autocorrelation and cross-correlation between surface pressures in different parts of the world. He discovered that there was swaying of pressure between the equatorial Pacific and equatorial Indian Ocean. He called this 'Southern Oscillation'. He called the swaying of pressure between Azores and Iceland as 'North Atlantic Oscillation' and similar oscillation in the northern Pacific Ocean as 'North Pacific Oscillation'. These three oscillations of surface pressure playa fundamental role in the variability of the earth's climate. -
A Pen Portrait of Sir Gilbert Walker
PEN PORTRAIT OF SIR GILBERT WALKER, CSI, MA, ScD, FRS by J M Walker University of Wales, Cardiff This pen portrait was published in the Royal Meteorological Society’s monthly magazine Weather in 1997 (Volume 52, No.7, pages 217-220) The choice of Gilbert Walker as Special Assistant to Sir John Eliot was surprising. Sir John was the Meteorological Reporter to the Government of India and Director-General of Indian Observatories. Walker was not a meteorologist. Hitherto, he had pursued an academic career as a mathematician at the University of Cambridge, where he had interested himself mainly in electromagnetism but had also taken an interest in the dynamics of spinning tops and projectiles. He was especially interested in boomerangs and had published an original and imaginative paper on them in 1897 (Phil. Trans Roy. Soc., A, 190, pp.23-42). His interest in this missile had begun in the late 1880s, during a visit to Australia, and his prowess in throwing boomerangs on the Cambridge Backs in his undergraduate days was such that his friends called him `Boomerang Walker'. In India, as Sir George Simpson recalled (Weather, 1959, 14, pp.67-68), Walker used to throw his boomerangs on Annandale, the only level piece of ground in Simla, "much to the interest of the Viceroy and his numerous military friends". Whilst at Cambridge, Walker came to be recognised as an expert on mathematical aspects of sport and games and by invitation wrote "Spiel und Sport", a scholarly article on billiards, ball games (particularly golf), boomerangs and bicycles which was published in the Enzyklopädie der mathematischen Wissenschaften (1900, 4, 9, pp.127-152).