Spectroscopic and Spectro-Astrometric Analysis of T Tauri Stars
Total Page:16
File Type:pdf, Size:1020Kb
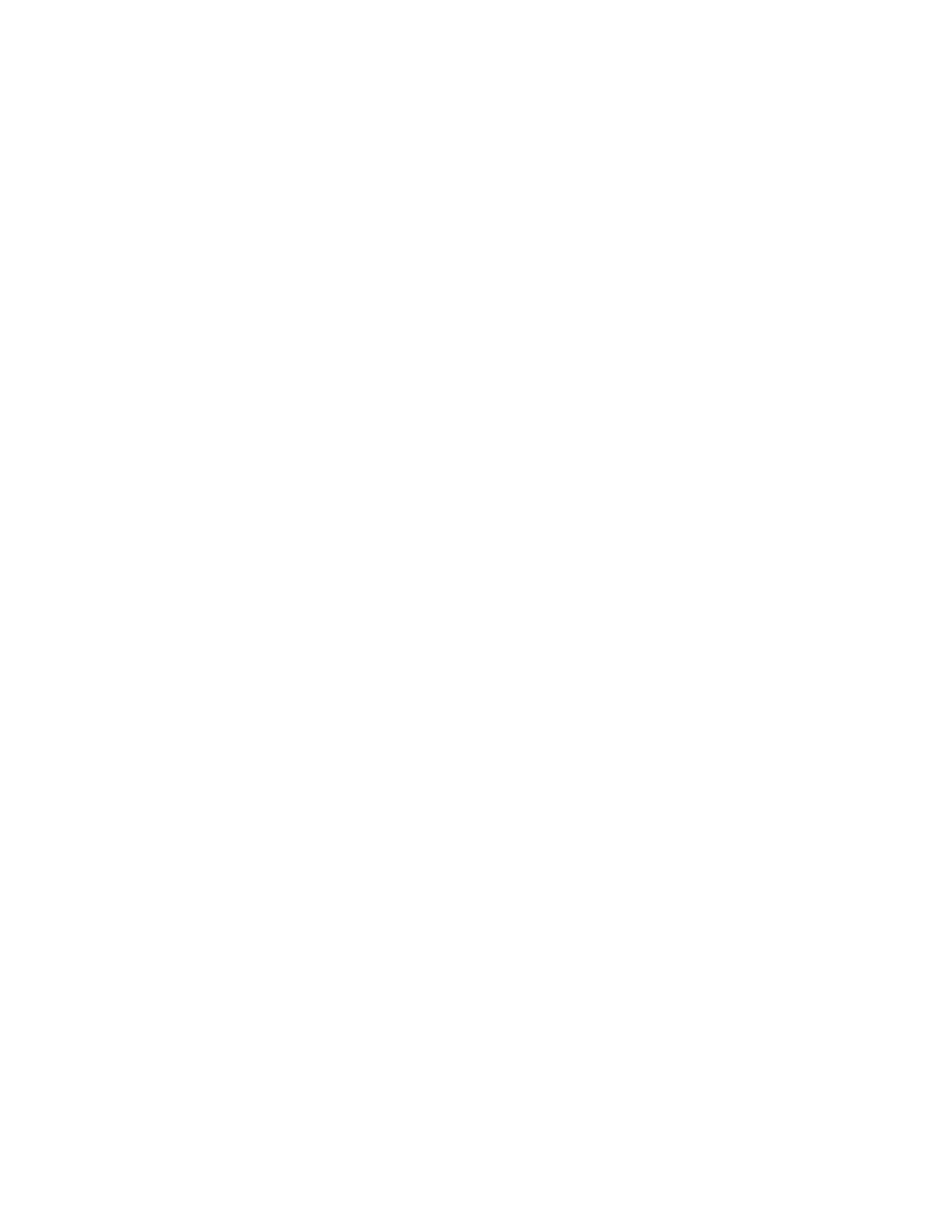
Load more
Recommended publications
-
Chemical Evolution of Protoplanetary Disks-The Effects of Viscous Accretion, Turbulent Mixing and Disk Winds
Draft version June 5, 2018 Preprint typeset using LATEX style emulateapj v. 11/10/09 CHEMICAL EVOLUTION OF PROTOPLANETARY DISKS – THE EFFECTS OF VISCOUS ACCRETION, TURBULENT MIXING AND DISK WINDS D. Heinzeller1 and H. Nomura Department of Astronomy, Graduate School of Science, Kyoto University, Kyoto 606-8502, Japan and C. Walsh and T. J. Millar Astrophysics Research Centre, School of Mathematics and Physics, Queen’s University Belfast, Belfast, BT7 1NN, UK Draft version June 5, 2018 ABSTRACT We calculate the chemical evolution of protoplanetary disks considering radial viscous accretion, vertical turbulent mixing and vertical disk winds. We study the effects on the disk chemical structure when different models for the formation of molecular hydrogen on dust grains are adopted. Our gas- phase chemistry is extracted from the UMIST Database for Astrochemistry (Rate06) to which we have added detailed gas-grain interactions. We use our chemical model results to generate synthetic near- and mid-infrared LTE line emission spectra and compare these with recent Spitzer observations. Our results show that if H2 formation on warm grains is taken into consideration, the H2O and OH abundances in the disk surface increase significantly. We find the radial accretion flow strongly influences the molecular abundances, with those in the cold midplane layers particularly affected. On the other hand, we show that diffusive turbulent mixing affects the disk chemistry in the warm molecular layers, influencing the line emission from the disk and subsequently improving agreement with observations. We find that NH3, CH3OH, C2H2 and sulphur-containing species are greatly enhanced by the inclusion of turbulent mixing. -
FY08 Technical Papers by GSMTPO Staff
AURA/NOAO ANNUAL REPORT FY 2008 Submitted to the National Science Foundation July 23, 2008 Revised as Complete and Submitted December 23, 2008 NGC 660, ~13 Mpc from the Earth, is a peculiar, polar ring galaxy that resulted from two galaxies colliding. It consists of a nearly edge-on disk and a strongly warped outer disk. Image Credit: T.A. Rector/University of Alaska, Anchorage NATIONAL OPTICAL ASTRONOMY OBSERVATORY NOAO ANNUAL REPORT FY 2008 Submitted to the National Science Foundation December 23, 2008 TABLE OF CONTENTS EXECUTIVE SUMMARY ............................................................................................................................. 1 1 SCIENTIFIC ACTIVITIES AND FINDINGS ..................................................................................... 2 1.1 Cerro Tololo Inter-American Observatory...................................................................................... 2 The Once and Future Supernova η Carinae...................................................................................................... 2 A Stellar Merger and a Missing White Dwarf.................................................................................................. 3 Imaging the COSMOS...................................................................................................................................... 3 The Hubble Constant from a Gravitational Lens.............................................................................................. 4 A New Dwarf Nova in the Period Gap............................................................................................................ -
RADIAL VELOCITIES in the ZODIACAL DUST CLOUD
A SURVEY OF RADIAL VELOCITIES in the ZODIACAL DUST CLOUD Brian Harold May Astrophysics Group Department of Physics Imperial College London Thesis submitted for the Degree of Doctor of Philosophy to Imperial College of Science, Technology and Medicine London · 2007 · 2 Abstract This thesis documents the building of a pressure-scanned Fabry-Perot Spectrometer, equipped with a photomultiplier and pulse-counting electronics, and its deployment at the Observatorio del Teide at Izaña in Tenerife, at an altitude of 7,700 feet (2567 m), for the purpose of recording high-resolution spectra of the Zodiacal Light. The aim was to achieve the first systematic mapping of the MgI absorption line in the Night Sky, as a function of position in heliocentric coordinates, covering especially the plane of the ecliptic, for a wide variety of elongations from the Sun. More than 250 scans of both morning and evening Zodiacal Light were obtained, in two observing periods – September-October 1971, and April 1972. The scans, as expected, showed profiles modified by components variously Doppler-shifted with respect to the unshifted shape seen in daylight. Unexpectedly, MgI emission was also discovered. These observations covered for the first time a span of elongations from 25º East, through 180º (the Gegenschein), to 27º West, and recorded average shifts of up to six tenths of an angstrom, corresponding to a maximum radial velocity relative to the Earth of about 40 km/s. The set of spectra obtained is in this thesis compared with predictions made from a number of different models of a dust cloud, assuming various distributions of dust density as a function of position and particle size, and differing assumptions about their speed and direction. -
Optical Spectroscopic Monitoring Observations of a T Tauri Star V409 Tau
International Journal of Astronomy and Astrophysics, 2019, 9, 321-334 http://www.scirp.org/journal/ijaa ISSN Online: 2161-4725 ISSN Print: 2161-4717 Optical Spectroscopic Monitoring Observations of a T Tauri Star V409 Tau Hinako Akimoto, Yoichi Itoh Nishi-Harima Astronomical Observatory, Center for Astronomy, University of Hyogo, Hyogo, Japan How to cite this paper: Akimoto, H. and Abstract Itoh, Y. (2019) Optical Spectroscopic Mon- itoring Observations of a T Tauri Star V409 We report the results of optical spectroscopic monitoring observations of a T Tau. International Journal of Astronomy Tauri star, V409 Tau. A previous photometric study indicated that this star and Astrophysics, 9, 321-334. experienced dimming events due to the obscuration of light from the central https://doi.org/10.4236/ijaa.2019.93023 star with a distorted circumstellar disk. We conducted medium-resolution (R Received: July 24, 2019 ~10,000) spectroscopic observations with 2-m Nayuta telescope at Ni- Accepted: September 16, 2019 shi-Harima Astronomical Observatory. Spectra were obtained in 18 nights Published: September 19, 2019 between November 2015 and March 2016. Several absorption lines such as Ca Copyright © 2019 by author(s) and I and Li, and the Hα emission line were confirmed in the spectra. The Ic-band Scientific Research Publishing Inc. magnitudes of V409 Tau changed by approximately 1 magnitude during the This work is licensed under the Creative observation epoch. The equivalent widths of the five absorption lines are Commons Attribution International License (CC BY 4.0). roughly constant despite changes in the Ic-band magnitudes. We conclude http://creativecommons.org/licenses/by/4.0/ that the light variation of the star is caused by the obscuration of light from Open Access the central star with a distorted circumstellar disk, based on the relationship between the equivalent widths of the absorption lines and the Ic-band magni- tudes. -
Optical Spectroscopic Monitoring Observations of a T Tauri Star V409
International Journal of Astronomy & Astrophysics, 2019, *,** Published Online **** 2019 in SciRes. http://www.scirp.org/journal/**** http://dx.doi.org/10.4236/****.2014.***** Optical Spectroscopic Monitoring Observations of a T Tauri Star V409 Tau Hinako Akimoto1 and Yoichi Itoh1 1 Nishi-Harima Astronomical Observatory, Center for Astronomy, University of Hyogo, 407-2 Nishigaichi, Sayo, Sayo, Hyogo 679-5313, Japan Email: [email protected] Received **** 2019 Copyright c 2019 by author(s) and Scientific Research Publishing Inc. This work is licensed under the Creative Commons Attribution International License (CC BY). http://creativecommons.org/licenses/by/4.0/ Abstract We report the results of optical spectroscopic monitoring observations of a T Tauri star, V409 Tau. A previous photometric study indicated that this star experienced dimming events due to the obscuration of light from the central star with a distorted circumstellar disk. We conducted medium-resolution (R ∼ 10000) spectroscopic observations with 2-m Nayuta telescope at Nishi-Harima Astronomical Observatory. Spectra were obtained in 18 nights between November 2015 and March 2016. Several absorption lines such as Ca I and Li, and the Hα emission line were confirmed in the spectra. The Ic-band magnitudes of V409 Tau changed by approximately 1 magnitude during the observation epoch. The equivalent widths of the five absorption lines are roughly constant despite changes in the Ic-band magnitudes. We conclude that the light variation of the star is caused by the obscuration of light from the central star with a distorted circumstellar disk, based on the relationship between the equivalent widths of the absorption lines and the Ic-band magnitudes. -
Discovery of Variable X-Ray Absorption in the Ctts AA Tauri J
A&A 462, L41–L44 (2007) Astronomy DOI: 10.1051/0004-6361:20066693 & c ESO 2007 Astrophysics Letter to the Editor Discovery of variable X-ray absorption in the cTTS AA Tauri J. H. M. M. Schmitt and J. Robrade Hamburger Sternwarte, Gojenbergsweg 112, 21029 Hamburg, Germany e-mail: [email protected] Received 3 November 2006 / Accepted 6 December 2006 ABSTRACT We present XMM-Newton X-ray and UV observations of the classical T Tauri star AA Tau covering almost two rotational periods where Prot ∼ 8.5 days. Clear, but uncorrelated variability is found at both wavebands. The variability observed at ∼2100 Å follows the previously known optical period. Spectral analysis of the X-ray data results in significant variability in the X-ray absorption such that the times of maximal X-ray absorption and UV extinction coincide. Placing the coronal emission in regions at low up to moderate magnetic latitudes and attribution of the variable X-ray absorption to accretion curtains and/or the disk warp provides a consistent physical picture. However, the derived X-ray absorption and optical extinction at times of maximal optical/UV brightness, i.e. outside occultation, are difficult to reconcile, requiring additional absorption in a disk wind or a peculiar dust grain distribution. Key words. X-rays: stars – stars: pre-main sequence – stars: coronae – stars: activity 1. Introduction warp leads to a partial occultation of AA Tau’s photosphere, thus causing the observed almost achromatic brightness variations. T Tauri stars (TTS) are very young low-mass stars evolving to- Polarization measurements (Ménard et al. -
Download This Article in PDF Format
A&A 531, A80 (2011) Astronomy DOI: 10.1051/0004-6361/201116757 & c ESO 2011 Astrophysics Stirring up the dust: a dynamical model for halo-like dust clouds in transitional disks S. Krijt1,2 and C. Dominik2,3 1 Leiden Observatory, Niels Bohrweg 2, 2333 CA Leiden, The Netherlands e-mail: [email protected] 2 Astronomical Institute “Anton Pannekoek”, University of Amsterdam, PO Box 94249, 1090 GE Amsterdam, The Netherlands 3 Department of Astrophysics, Radboud University Nijmegen, PO Box 9010, 6500 GL Nijmegen, The Netherlands Received 21 February 2011 / Accepted 1 May 2011 ABSTRACT Context. A small number of young stellar objects show signs of a halo-like structure of optically thin dust, in addition to a circum- stellar disk. This halo or torus is located within a few AU of the star, but its origin has not yet been understood. Aims. A dynamically excited cloud of planetesimals colliding to eventually form dust could produce such a structure. The cause of the dynamical excitation could be one or more planets, perhaps on eccentric orbits, or a migrating planet. This work investigates an inwardly migrating planet that is dynamically scattering planetesimals as a possible cause for the observed structures. If this mecha- nism is responsible, the observed halo-like structure could be used to infer the existence of planets in these systems. Methods. We present analytical estimates on the maximum inclination reached owing to dynamical interactions between planetesi- mals and a migrating planet. In addition, a symplectic integrator is used to simulate the effect of a migrating planet on a population of planetesimals. -
Binocular Double Star Logbook
Astronomical League Binocular Double Star Club Logbook 1 Table of Contents Alpha Cassiopeiae 3 14 Canis Minoris Sh 251 (Oph) Psi 1 Piscium* F Hydrae Psi 1 & 2 Draconis* 37 Ceti Iota Cancri* 10 Σ2273 (Dra) Phi Cassiopeiae 27 Hydrae 40 & 41 Draconis* 93 (Rho) & 94 Piscium Tau 1 Hydrae 67 Ophiuchi 17 Chi Ceti 35 & 36 (Zeta) Leonis 39 Draconis 56 Andromedae 4 42 Leonis Minoris Epsilon 1 & 2 Lyrae* (U) 14 Arietis Σ1474 (Hya) Zeta 1 & 2 Lyrae* 59 Andromedae Alpha Ursae Majoris 11 Beta Lyrae* 15 Trianguli Delta Leonis Delta 1 & 2 Lyrae 33 Arietis 83 Leonis Theta Serpentis* 18 19 Tauri Tau Leonis 15 Aquilae 21 & 22 Tauri 5 93 Leonis OΣΣ178 (Aql) Eta Tauri 65 Ursae Majoris 28 Aquilae Phi Tauri 67 Ursae Majoris 12 6 (Alpha) & 8 Vul 62 Tauri 12 Comae Berenices Beta Cygni* Kappa 1 & 2 Tauri 17 Comae Berenices Epsilon Sagittae 19 Theta 1 & 2 Tauri 5 (Kappa) & 6 Draconis 54 Sagittarii 57 Persei 6 32 Camelopardalis* 16 Cygni 88 Tauri Σ1740 (Vir) 57 Aquilae Sigma 1 & 2 Tauri 79 (Zeta) & 80 Ursae Maj* 13 15 Sagittae Tau Tauri 70 Virginis Theta Sagittae 62 Eridani Iota Bootis* O1 (30 & 31) Cyg* 20 Beta Camelopardalis Σ1850 (Boo) 29 Cygni 11 & 12 Camelopardalis 7 Alpha Librae* Alpha 1 & 2 Capricorni* Delta Orionis* Delta Bootis* Beta 1 & 2 Capricorni* 42 & 45 Orionis Mu 1 & 2 Bootis* 14 75 Draconis Theta 2 Orionis* Omega 1 & 2 Scorpii Rho Capricorni Gamma Leporis* Kappa Herculis Omicron Capricorni 21 35 Camelopardalis ?? Nu Scorpii S 752 (Delphinus) 5 Lyncis 8 Nu 1 & 2 Coronae Borealis 48 Cygni Nu Geminorum Rho Ophiuchi 61 Cygni* 20 Geminorum 16 & 17 Draconis* 15 5 (Gamma) & 6 Equulei Zeta Geminorum 36 & 37 Herculis 79 Cygni h 3945 (CMa) Mu 1 & 2 Scorpii Mu Cygni 22 19 Lyncis* Zeta 1 & 2 Scorpii Epsilon Pegasi* Eta Canis Majoris 9 Σ133 (Her) Pi 1 & 2 Pegasi Δ 47 (CMa) 36 Ophiuchi* 33 Pegasi 64 & 65 Geminorum Nu 1 & 2 Draconis* 16 35 Pegasi Knt 4 (Pup) 53 Ophiuchi Delta Cephei* (U) The 28 stars with asterisks are also required for the regular AL Double Star Club. -
Hans Moritz Günther
page 1 of 3 Hans Moritz G¨unther - List of publications Refereed publications First author publications 16. YSOVAR: Mid-infrared Variability in the Star-forming Region Lynds 1688 H. M. G¨unther, A. M. Cody, K. R. Covey, L. A. Hillenbrand, P. Plavchan, K. Poppenhaeger, L. M. Rebull, J. R. Stauffer, S. J. Wolk, L. Allen, A. Bayo, R. A. Gutermuth, J. L. Hora, H. Y. A. Meng, M. Morales-Calder´on,J. R. Parks, Insoek Song (2014), AJ 148, 122 15. Recollimation Boundary Layers as X-Ray Sources in Young Stellar Jets H. M. G¨unther, Zhi-Yun Li, P. C. Schneider (2014), ApJ, 795, 51 14. MN Lup: X-rays from a Weakly Accreting T Tauri Star H. M. G¨unther, U. Wolter, J. Robrade, S. J. Wolk (2013), ApJ 771, 70 13. The evolution of the jet from the Herbig Ae Star HD 163296 in the last decade H. M. G¨unther, P. C. Schneider, Z.-Y. Li (2013), A&A 552, 142 12. Accretion, winds and outflows in young stars H. M. G¨unther (2013), AN 334, 67 11. IRAS 20050+2720: Anatomy of a Young Stellar Cluster H. M. G¨unther, S. J. Wolk, B. Spitzbart, R. A. Gutermuth, J. Forbrich, N. J. Wright, L. Allen, T. L. Bourke, S. T. Megeath, J. L. Pipher (2012), AJ 144, 101 10. Soft Coronal X-Rays from β Pictoris H. M. G¨unther, S. J. Wolk, J. J. Drake, C. M. Lisse,J. Robrade, J. H. M. M. Schmitt (2012), ApJ 750, 78 9. Accretion, jets and winds: High-energy emission from young stellar objects H. -
Dust and Molecular Shells in Asymptotic Giant Branch Stars⋆⋆⋆⋆⋆⋆
A&A 545, A56 (2012) Astronomy DOI: 10.1051/0004-6361/201118150 & c ESO 2012 Astrophysics Dust and molecular shells in asymptotic giant branch stars,, Mid-infrared interferometric observations of R Aquilae, R Aquarii, R Hydrae, W Hydrae, and V Hydrae R. Zhao-Geisler1,2,†, A. Quirrenbach1, R. Köhler1,3, and B. Lopez4 1 Zentrum für Astronomie der Universität Heidelberg (ZAH), Landessternwarte, Königstuhl 12, 69120 Heidelberg, Germany e-mail: [email protected] 2 National Taiwan Normal University, Department of Earth Sciences, 88 Sec. 4, Ting-Chou Rd, Wenshan District, Taipei, 11677 Taiwan, ROC 3 Max-Planck-Institut für Astronomie, Königstuhl 17, 69120 Heidelberg, Germany 4 Laboratoire J.-L. Lagrange, Université de Nice Sophia-Antipolis et Observatoire de la Cˆote d’Azur, BP 4229, 06304 Nice Cedex 4, France Received 26 September 2011 / Accepted 21 June 2012 ABSTRACT Context. Asymptotic giant branch (AGB) stars are one of the largest distributors of dust into the interstellar medium. However, the wind formation mechanism and dust condensation sequence leading to the observed high mass-loss rates have not yet been constrained well observationally, in particular for oxygen-rich AGB stars. Aims. The immediate objective in this work is to identify molecules and dust species which are present in the layers above the photosphere, and which have emission and absorption features in the mid-infrared (IR), causing the diameter to vary across the N-band, and are potentially relevant for the wind formation. Methods. Mid-IR (8–13 μm) interferometric data of four oxygen-rich AGB stars (R Aql, R Aqr, R Hya, and W Hya) and one carbon- rich AGB star (V Hya) were obtained with MIDI/VLTI between April 2007 and September 2009. -
The UV Perspective of Low-Mass Star Formation
galaxies Review The UV Perspective of Low-Mass Star Formation P. Christian Schneider 1,* , H. Moritz Günther 2 and Kevin France 3 1 Hamburger Sternwarte, University of Hamburg, 21029 Hamburg, Germany 2 Massachusetts Institute of Technology, Kavli Institute for Astrophysics and Space Research; Cambridge, MA 02109, USA; [email protected] 3 Department of Astrophysical and Planetary Sciences Laboratory for Atmospheric and Space Physics, University of Colorado, Denver, CO 80203, USA; [email protected] * Correspondence: [email protected] Received: 16 January 2020; Accepted: 29 February 2020; Published: 21 March 2020 Abstract: The formation of low-mass (M? . 2 M ) stars in molecular clouds involves accretion disks and jets, which are of broad astrophysical interest. Accreting stars represent the closest examples of these phenomena. Star and planet formation are also intimately connected, setting the starting point for planetary systems like our own. The ultraviolet (UV) spectral range is particularly suited for studying star formation, because virtually all relevant processes radiate at temperatures associated with UV emission processes or have strong observational signatures in the UV range. In this review, we describe how UV observations provide unique diagnostics for the accretion process, the physical properties of the protoplanetary disk, and jets and outflows. Keywords: star formation; ultraviolet; low-mass stars 1. Introduction Stars form in molecular clouds. When these clouds fragment, localized cloud regions collapse into groups of protostars. Stars with final masses between 0.08 M and 2 M , broadly the progenitors of Sun-like stars, start as cores deeply embedded in a dusty envelope, where they can be seen only in the sub-mm and far-IR spectral windows (so-called class 0 sources). -
Annual Report ESO Staff Papers 2018
ESO Staff Publications (2018) Peer-reviewed publications by ESO scientists The ESO Library maintains the ESO Telescope Bibliography (telbib) and is responsible for providing paper-based statistics. Publications in refereed journals based on ESO data (2018) can be retrieved through telbib: ESO data papers 2018. Access to the database for the years 1996 to present as well as an overview of publication statistics are available via http://telbib.eso.org and from the "Basic ESO Publication Statistics" document. Papers that use data from non-ESO telescopes or observations obtained with hosted telescopes are not included. The list below includes papers that are (co-)authored by ESO authors, with or without use of ESO data. It is ordered alphabetically by first ESO-affiliated author. Gravity Collaboration, Abuter, R., Amorim, A., Bauböck, M., Shajib, A.J., Treu, T. & Agnello, A., 2018, Improving time- Berger, J.P., Bonnet, H., Brandner, W., Clénet, Y., delay cosmography with spatially resolved kinematics, Coudé Du Foresto, V., de Zeeuw, P.T., et al. , 2018, MNRAS, 473, 210 [ADS] Detection of orbital motions near the last stable circular Treu, T., Agnello, A., Baumer, M.A., Birrer, S., Buckley-Geer, orbit of the massive black hole SgrA*, A&A, 618, L10 E.J., Courbin, F., Kim, Y.J., Lin, H., Marshall, P.J., Nord, [ADS] B., et al. , 2018, The STRong lensing Insights into the Gravity Collaboration, Abuter, R., Amorim, A., Anugu, N., Dark Energy Survey (STRIDES) 2016 follow-up Bauböck, M., Benisty, M., Berger, J.P., Blind, N., campaign - I. Overview and classification of candidates Bonnet, H., Brandner, W., et al.