A Unique Structural and Dynamic Feature of Aspartic Protease
Total Page:16
File Type:pdf, Size:1020Kb
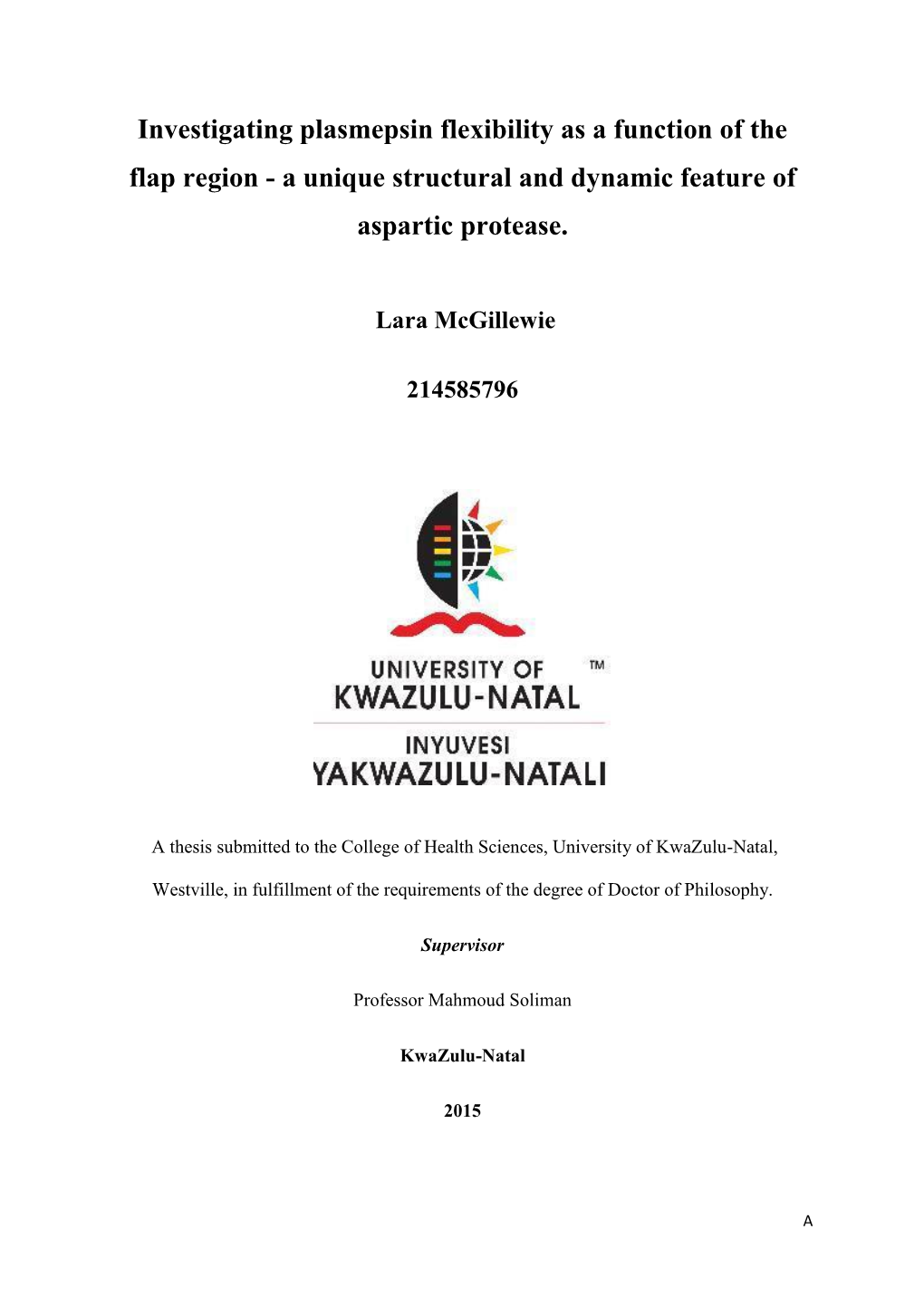
Load more
Recommended publications
-
Membrane Proteins • Cofactors – Plimstex • Membranes • Dna • Small Molecules/Gas • Large Complexes
Structural mass spectrometry hydrogen/deuterium exchange Petr Man Structural Biology and Cell Signalling Institute of Microbiology, Czech Academy of Sciences Structural biology methods Low-resolution methods High-resolution methods Rigid SAXS IR Raman CD ITC MST Cryo-EM AUC SPR MS X-ray crystallography Chemical cross-linking H/D exchange Native ESI + ion mobility Oxidative labelling Small Large NMR Dynamic Structural biology approaches Simple MS, quantitative MS Cross-linking, top-down, native MS+dissociation native MS+ion mobility Cross-linking Structural MS What can we get using mass spectrometry IM – ion mobility CXL – chemical cross-linking AP – afinity purification OFP – oxidative footprinting HDX – hydrogen/deuterium exchange ISOTOPE EXCHANGE IN PROTEINS 1H 2H 3H occurence [%] 99.988 0.0115 trace 5 …Kaj Ulrik Linderstrøm-Lang „Cartesian diver“ Proteins are migrating in tubes with density gradient until they stop at the point where the densities are equal 1H 2H 3H % 99.9885 0.0115 trace density [g/cm3] 1.000 1.106 1.215 Methods of detection IR: β-: NMR: 1 n = 1.6749 × 10-27 kg MS: 1H 2H 3H výskyt% [%] 99.9885 0.0115 trace hustotadensity vody [g/cm [g/cm3] 3] 1.000 1.106 1.215 jadernýspinspin ½+ 1+ ½+ mass [u] 1.00783 2.01410 3.01605 Factors affecting H/D exchange hydrogen bonding solvent accessibility Factors affecting H/D exchange Side chains (acidity, steric shielding) Bai et al.: Proteins (1993) Glasoe, Long: J. Phys. Chem. (1960) Factors affecting H/D exchange – side chain effects Inductive effect – electron density is Downward shift due to withdrawn from peptide steric hindrance effect of bond (S, O). -
Serine Proteases with Altered Sensitivity to Activity-Modulating
(19) & (11) EP 2 045 321 A2 (12) EUROPEAN PATENT APPLICATION (43) Date of publication: (51) Int Cl.: 08.04.2009 Bulletin 2009/15 C12N 9/00 (2006.01) C12N 15/00 (2006.01) C12Q 1/37 (2006.01) (21) Application number: 09150549.5 (22) Date of filing: 26.05.2006 (84) Designated Contracting States: • Haupts, Ulrich AT BE BG CH CY CZ DE DK EE ES FI FR GB GR 51519 Odenthal (DE) HU IE IS IT LI LT LU LV MC NL PL PT RO SE SI • Coco, Wayne SK TR 50737 Köln (DE) •Tebbe, Jan (30) Priority: 27.05.2005 EP 05104543 50733 Köln (DE) • Votsmeier, Christian (62) Document number(s) of the earlier application(s) in 50259 Pulheim (DE) accordance with Art. 76 EPC: • Scheidig, Andreas 06763303.2 / 1 883 696 50823 Köln (DE) (71) Applicant: Direvo Biotech AG (74) Representative: von Kreisler Selting Werner 50829 Köln (DE) Patentanwälte P.O. Box 10 22 41 (72) Inventors: 50462 Köln (DE) • Koltermann, André 82057 Icking (DE) Remarks: • Kettling, Ulrich This application was filed on 14-01-2009 as a 81477 München (DE) divisional application to the application mentioned under INID code 62. (54) Serine proteases with altered sensitivity to activity-modulating substances (57) The present invention provides variants of ser- screening of the library in the presence of one or several ine proteases of the S1 class with altered sensitivity to activity-modulating substances, selection of variants with one or more activity-modulating substances. A method altered sensitivity to one or several activity-modulating for the generation of such proteases is disclosed, com- substances and isolation of those polynucleotide se- prising the provision of a protease library encoding poly- quences that encode for the selected variants. -
Protease Inhibition—An Established Strategy to Combat Infectious Diseases
International Journal of Molecular Sciences Review Protease Inhibition—An Established Strategy to Combat Infectious Diseases Daniel Sojka 1,* , Pavla Šnebergerová 1,2 and Luïse Robbertse 1 1 Biology Centre, Institute of Parasitology, Academy of Sciences of the Czech Republic, Branišovská 1160/31, CZ-37005 Ceskˇ é Budˇejovice,Czech Republic; [email protected] (P.Š.); [email protected] (L.R.) 2 Faculty of Science, University of South Bohemia in Ceskˇ é Budˇejovice,Branišovská 1760c, CZ-37005 Ceskˇ é Budˇejovice,Czech Republic * Correspondence: [email protected] Abstract: Therapeutic agents with novel mechanisms of action are urgently needed to counter the emergence of drug-resistant infections. Several decades of research into proteases of disease agents have revealed enzymes well suited for target-based drug development. Among them are the three recently validated proteolytic targets: proteasomes of the malarial parasite Plasmodium falciparum, aspartyl proteases of P. falciparum (plasmepsins) and the Sars-CoV-2 viral proteases. Despite some unfulfilled expectations over previous decades, the three reviewed targets clearly demonstrate that selective protease inhibitors provide effective therapeutic solutions for the two most impacting infectious diseases nowadays—malaria and COVID-19. Keywords: protease; parasites; inhibition; therapy; infectious diseases Citation: Sojka, D.; Šnebergerová, P.; Robbertse, L. Protease Inhibition—An 1. Introduction Established Strategy to Combat Infectious diseases, along with starvation, limited water resources and the lack of Infectious Diseases. Int. J. Mol. Sci. shelter, are among the main factors threatening the health and prosperity of the world’s 2021, 22, 5762. https://doi.org/ growing human population. Significant proportions of infectious diseases are caused by 10.3390/ijms22115762 parasites [1], the most common human infections being toxoplasmosis, ascariasis, ancy- lostomiasis and trichomoniasis. -
Disulfide Linkages in Plasmodium Falciparum Plasmepsin-I Are Essential Elements for Its Processing Activity and Multi-Milligram Recombinant Production Yield
Disulfide Linkages in Plasmodium falciparum Plasmepsin-I Are Essential Elements for Its Processing Activity and Multi-Milligram Recombinant Production Yield Sirisak Lolupiman1, Pilaiwan Siripurkpong2, Jirundon Yuvaniyama1* 1 Department of Biochemistry and Center for Excellence in Protein Structure and Function, Faculty of Science, Mahidol University, Bangkok, Thailand, 2 Department of Medical Technology, Faculty of Allied Health Sciences, Thammasat University, Pathumthani, Thailand Abstract Plasmodium falciparum plasmepsin-I (PM-I) has been considered a potential drug target for the parasite that causes fatal malaria in human. Determination of PM-I structures for rational design of its inhibitors is hindered by the difficulty in obtaining large quantity of soluble enzyme. Nearly all attempts for its heterologous expression in Escherichia coli result in the production of insoluble proteins in both semi-pro-PM-I and its truncated form, and thus require protein refolding. Moreover, the yields of purified, soluble PM-I from all reported studies are very limited. Exclusion of truncated semi-pro-PM-I expression in E. coli C41(DE3) is herein reported. We also show that the low preparation yield of purified semi-pro-PM-I with autoprocessing ability is mainly a result of structural instability of the refolded enzyme in acidic conditions due to incomplete formation of disulfide linkages. Upon formation of at least one of the two natural disulfide bonds, nearly all of the refolded semi-pro-PM-I could be activated to its mature form. A significantly improved yield of 10 mg of semi-pro-PM-I per liter of culture, which resulted in 6–8 mg of the mature PM-I, was routinely obtained using this strategy. -
Data-Mining Approaches Reveal Hidden Families of Proteases in The
Downloaded from genome.cshlp.org on October 5, 2021 - Published by Cold Spring Harbor Laboratory Press Letter Data-Mining Approaches Reveal Hidden Families of Proteases in the Genome of Malaria Parasite Yimin Wu,1,4 Xiangyun Wang,2 Xia Liu,1 and Yufeng Wang3,5 1Department of Protistology, American Type Culture Collection, Manassas, Virginia 20110, USA; 2EST Informatics, Astrazeneca Pharmaceuticals, Wilmington, Delaware 19810, USA; 3Department of Bioinformatics, American Type Culture Collection, Manassas, Virginia 20110, USA The search for novel antimalarial drug targets is urgent due to the growing resistance of Plasmodium falciparum parasites to available drugs. Proteases are attractive antimalarial targets because of their indispensable roles in parasite infection and development,especially in the processes of host e rythrocyte rupture/invasion and hemoglobin degradation. However,to date,only a small number of protease s have been identified and characterized in Plasmodium species. Using an extensive sequence similarity search,we have identifi ed 92 putative proteases in the P. falciparum genome. A set of putative proteases including calpain,metacaspase,and s ignal peptidase I have been implicated to be central mediators for essential parasitic activity and distantly related to the vertebrate host. Moreover,of the 92,at least 88 have been demonstrate d to code for gene products at the transcriptional levels,based upon the microarray and RT-PCR results,an d the publicly available microarray and proteomics data. The present study represents an initial effort to identify a set of expressed,active,and essential proteases as targets for inhibitor-based drug design. [Supplemental material is available online at www.genome.org.] Malaria remains one of the most dangerous infectious diseases metalloprotease (falcilysin; Eggleson et al. -
Understanding the Structural Basis of Substrate Recognition By
www.nature.com/scientificreports OPEN Understanding the structural basis of substrate recognition by Plasmodium falciparum plasmepsin V Received: 02 November 2015 Accepted: 20 July 2016 to aid in the design of potent Published: 17 August 2016 inhibitors Rajiv K. Bedi1,*, Chandan Patel2,*, Vandana Mishra1, Huogen Xiao3, Rickey Y. Yada4 & Prasenjit Bhaumik1 Plasmodium falciparum plasmepsin V (PfPMV) is an essential aspartic protease required for parasite survival, thus, considered as a potential drug target. This study reports the first detailed structural analysis and molecular dynamics simulation of PfPMV as an apoenzyme and its complexes with the substrate PEXEL as well as with the inhibitor saquinavir. The presence of pro-peptide in PfPMV may not structurally hinder the formation of a functionally competent catalytic active site. The structure of PfPMV-PEXEL complex shows that the unique positions of Glu179 and Gln222 are responsible for providing the specificity of PEXEL substrate with arginine at P3 position. The structural analysis also reveals that the S4 binding pocket in PfPMV is occupied by Ile94, Ala98, Phe370 and Tyr472, and therefore, does not allow binding of pepstatin, a potent inhibitor of most pepsin-like aspartic proteases. Among the screened inhibitors, the HIV-1 protease inhibitors and KNI compounds have higher binding affinities for PfPMV with saquinavir having the highest value. The presence of a flexible group at P2 and a bulky hydrophobic group at P3 position of the inhibitor is preferred in the PfPMV substrate binding pocket. Results from the present study will aid in the design of potent inhibitors of PMV. Malaria is an infectious disease that is responsible for causing illness in an estimated 200 to 500 million people and results in an annual mortality of 1 to 2 million persons1. -
Nepenthesin from Monkey Cups for Hydrogen/Deuterium Exchange Mass Spectrometry
University of Calgary PRISM: University of Calgary's Digital Repository Cumming School of Medicine Cumming School of Medicine Research & Publications 2013-02 Nepenthesin from monkey cups for hydrogen/deuterium exchange mass spectrometry. Rey, Martial; Yang, Menglin; Burns, Kyle M.; Yu, Yaping; Lees-Miller, Susan P.; Schriemer, David C. MOLECULAR & CELLULAR PROTEOMICS Rey, M., Yang, M., Burns, K. M., Yu, Y., Lees-Miller, S. P., & Schriemer, D. C. (2013). Nepenthesin from monkey cups for hydrogen/deuterium exchange mass spectrometry. Molecular and Cellular Proteomics, 12(2), 464-472. doi:10.1074/mcp.M112.025221 http://hdl.handle.net/1880/52223 journal article Downloaded from PRISM: https://prism.ucalgary.ca Research © 2013 by The American Society for Biochemistry and Molecular Biology, Inc. This paper is available on line at http://www.mcponline.org Nepenthesin from Monkey Cups for Hydrogen/ Deuterium Exchange Mass Spectrometry*□S Martial Rey‡, Menglin Yang‡, Kyle M. Burns‡, Yaping Yu‡, Susan P. Lees-Miller‡, and David C. Schriemer‡§ Studies of protein dynamics, structure and interactions fication and characterization, but in the last several years it using hydrogen/deuterium exchange mass spectrometry has also become a powerful tool for interrogating protein (HDX-MS) have sharply increased over the past 5–10 structure and dynamics (1, 2). Solution-phase hydrogen/deu- years. The predominant technology requires fast diges- terium exchange (HDX)1, when coupled with mass spectrom- tion at pH 2–3 to retain deuterium label. Pepsin is used etry (MS), provides rich sets of data that can be mined to almost exclusively, but it provides relatively low efficiency extract structural and dynamic parameters from proteins (3– under the constraints of the experiment, and a selectivity 7). -
Handbook of Proteolytic Enzymes Second Edition Volume 1 Aspartic and Metallo Peptidases
Handbook of Proteolytic Enzymes Second Edition Volume 1 Aspartic and Metallo Peptidases Alan J. Barrett Neil D. Rawlings J. Fred Woessner Editor biographies xxi Contributors xxiii Preface xxxi Introduction ' Abbreviations xxxvii ASPARTIC PEPTIDASES Introduction 1 Aspartic peptidases and their clans 3 2 Catalytic pathway of aspartic peptidases 12 Clan AA Family Al 3 Pepsin A 19 4 Pepsin B 28 5 Chymosin 29 6 Cathepsin E 33 7 Gastricsin 38 8 Cathepsin D 43 9 Napsin A 52 10 Renin 54 11 Mouse submandibular renin 62 12 Memapsin 1 64 13 Memapsin 2 66 14 Plasmepsins 70 15 Plasmepsin II 73 16 Tick heme-binding aspartic proteinase 76 17 Phytepsin 77 18 Nepenthesin 85 19 Saccharopepsin 87 20 Neurosporapepsin 90 21 Acrocylindropepsin 9 1 22 Aspergillopepsin I 92 23 Penicillopepsin 99 24 Endothiapepsin 104 25 Rhizopuspepsin 108 26 Mucorpepsin 11 1 27 Polyporopepsin 113 28 Candidapepsin 115 29 Candiparapsin 120 30 Canditropsin 123 31 Syncephapepsin 125 32 Barrierpepsin 126 33 Yapsin 1 128 34 Yapsin 2 132 35 Yapsin A 133 36 Pregnancy-associated glycoproteins 135 37 Pepsin F 137 38 Rhodotorulapepsin 139 39 Cladosporopepsin 140 40 Pycnoporopepsin 141 Family A2 and others 41 Human immunodeficiency virus 1 retropepsin 144 42 Human immunodeficiency virus 2 retropepsin 154 43 Simian immunodeficiency virus retropepsin 158 44 Equine infectious anemia virus retropepsin 160 45 Rous sarcoma virus retropepsin and avian myeloblastosis virus retropepsin 163 46 Human T-cell leukemia virus type I (HTLV-I) retropepsin 166 47 Bovine leukemia virus retropepsin 169 48 -
1 Peptide Therapeutics
Universal Free E-Book Store Donate Us, In order to keep our Service Alive, We have to pay for placing files (Abstracts, Books, Literature & Software) on File Hosting Servers, Your donations will make our process of payment a bit easier, Please use any one of the Payment Gateway for Donation. Never matter what amount you donate (10’s or 100’s or 1000’s). Universal Free E-Book Store Universal Free E-Book Store PEPTIDE CHEMISTRY AND DRUG DESIGN Universal Free E-Book Store Universal Free E-Book Store PEPTIDE CHEMISTRY AND DRUG DESIGN Edited by BEN M. DUNN Universal Free E-Book Store Copyright © 2015 by John Wiley & Sons, Inc. All rights reserved Published by John Wiley & Sons, Inc., Hoboken, New Jersey Published simultaneously in Canada No part of this publication may be reproduced, stored in a retrieval system, or transmitted in any form or by any means, electronic, mechanical, photocopying, recording, scanning, or otherwise, except as permitted under Section 107 or 108 of the 1976 United States Copyright Act, without either the prior written permission of the Publisher, or authorization through payment of the appropriate per-copy fee to the Copyright Clearance Center, Inc., 222 Rosewood Drive, Danvers, MA 01923, (978) 750-8400, fax (978) 750-4470, or on the web at www.copyright.com. Requests to the Publisher for permission should be addressed to the Permissions Department, John Wiley & Sons, Inc., 111 River Street, Hoboken, NJ 07030, (201) 748-6011, fax (201) 748-6008, or online at http://www.wiley.com/go/permissions. Limit of Liability/Disclaimer of Warranty: While the publisher and author have used their best efforts in preparing this book, they make no representations or warranties with respect to the accuracy or completeness of the contents of this book and specifically disclaim any implied warranties of merchantability or fitness for a particular purpose. -
University of Alberta Structural Studies Of
UNIVERSITY OF ALBERTA STRUCTURAL STUDIES OF MALARIAL ASPARTIC PROTEINASE ACTIVATION NINA KHAZANOVICH BERNSTEIN O A THESIS SUBMITTED TO THE FACULTY OF GRADUATE STUDES AND RESEARCH IN PARTIAL FULFILMENT OF THE REQUIREMENTS FOR THE DEGREE OF DOCTOR OF PHILOSOPHY DEPARTMENT OF BIOCHEMISTRY EDMONTON, ALBERTA SPFüNG, 2000 National tibraiy Bibliothbque nationale 141 dc-da du Canada A uisitionsarid Acquisitions et B8o9raphic Services services bibliographiques 385 ~eüingbmçtreet 395, rue WeUingtori Ottawa ON KIA ON4 OttawaON KIAW Canada Canada The author has granted a non- L'auteur a accordé une licence non exclusive licence allowing the exclusive permettant à la Natioaal Library of Canada to Bibliothèque nationale du Canada de reproduce, loan, distriiute or sell reproduire, prêter, distri'buer ou copies of this thesis in microform, vendre des copies de cette thèse sous paper or electronic formats. la forme de microfiche/nlm, de reproduction sur papier ou sur format électronique. The author retains ownership of the L'auteur conserve la propriété du copyright in this thesis. Neither the droit d'auteur qui protège cette thèse. thesis nor substantial extracts fiom it Ni la thèse ni des extraits substantiels may be printed or otherwise de celle-ci ne doivent être imprimes reproduced without the author's ou autrement reproduits sans son permission. autorisation. Dedicated to my parents. Abstract Malaria is a complex and devastating disease that is a major public heaith problem in many areas of the world During the blood stage of the malaria infection, the malaria parasite, Plasmodium, lives in the human host's erythrocytes, where it digests large quantities of hernoglobin as a source of nutrients. -
Overexpression of Plasmepsin II and Plasmepsin III Does Not Directly Cause Reduction in Plasmodium Falciparum Sensitivity to Artesunate, Chloroquine T and Piperaquine
IJP: Drugs and Drug Resistance 9 (2019) 16–22 Contents lists available at ScienceDirect IJP: Drugs and Drug Resistance journal homepage: www.elsevier.com/locate/ijpddr Overexpression of plasmepsin II and plasmepsin III does not directly cause reduction in Plasmodium falciparum sensitivity to artesunate, chloroquine T and piperaquine Duangkamon Loesbanluechaia,b, Namfon Kotanana, Cristina de Cozarc, Theerarat Kochakarna, Megan R. Ansbrod,e, Kesinee Chotivanichf,g, Nicholas J. Whiteg,h, Prapon Wilairati, ∗∗ Marcus C.S. Leee, Francisco Javier Gamoc, Laura Maria Sanzc, Thanat Chookajorna, , ∗ Krittikorn Kümpornsine, a Genomics and Evolutionary Medicine Unit (GEM), Centre of Excellence in Malaria Research, Faculty of Tropical Medicine, Mahidol University, Bangkok, 10400, Thailand b Molecular Medicine Program, Multidisciplinary Unit, Faculty of Science, Mahidol University, Bangkok, 10400, Thailand c Tres Cantos Medicine Development Campus, GlaxoSmithKline, Parque Tecnológico de Madrid, Tres Cantos, 28760, Spain d Laboratory of Malaria and Vector Research, National Institute of Allergy and Infectious Diseases, National Institutes of Health, Rockville, MD, 20852, USA e Parasites and Microbes Programme, Wellcome Sanger Institute, Wellcome Genome Campus, Hinxton, CB10 1SA, United Kingdom f Department of Clinical Tropical Medicine, Faculty of Tropical Medicine, Mahidol University, Bangkok, 10400, Thailand g Mahidol-Oxford Tropical Medicine Research Unit, Faculty of Tropical Medicine, Mahidol University, Bangkok, 10400, Thailand h Centre for Tropical Medicine and Global Health, Nuffield Department of Clinical Medicine, Churchill Hospital, Oxford, OX3 7LJ, United Kingdom i Department of Biochemistry, Faculty of Science, Mahidol University, Bangkok, 10400, Thailand ARTICLE INFO ABSTRACT Keywords: Artemisinin derivatives and their partner drugs in artemisinin combination therapies (ACTs) have played a Artemisinin pivotal role in global malaria mortality reduction during the last two decades. -
Hemoglobin-Degrading, Aspartic Proteases of Blood-Feeding Parasites SUBSTRATE SPECIFICITY REVEALED by HOMOLOGY MODELS*
THE JOURNAL OF BIOLOGICAL CHEMISTRY Vol. 276, No. 42, Issue of October 19, pp. 38844–38851, 2001 © 2001 by The American Society for Biochemistry and Molecular Biology, Inc. Printed in U.S.A. Hemoglobin-degrading, Aspartic Proteases of Blood-feeding Parasites SUBSTRATE SPECIFICITY REVEALED BY HOMOLOGY MODELS* Received for publication, March 2, 2001, and in revised form, August 6, 2001 Published, JBC Papers in Press, August 8, 2001, DOI 10.1074/jbc.M101934200 Ross I. Brinkworth‡, Paul Prociv§, Alex Loukas¶, and Paul J. Brindleyʈ** From the ‡Institute of Molecular Biosciences and §Department of Microbiology and Parasitology, University of Queensland, Brisbane, Queensland 4072, Australia, ¶Division of Infectious Diseases and Immunology, Queensland Institute of Medical Research, Brisbane, Queensland 4029, Australia, and ʈDepartment of Tropical Medicine, School of Public Health and Tropical Medicine, Tulane University, New Orleans, Louisiana 70112 Blood-feeding parasites, including schistosomes, hook- and mortality (1). Although phylogenetically unrelated, these worms, and malaria parasites, employ aspartic pro- parasites all share the same food source; they are obligate blood teases to make initial or early cleavages in ingested host feeders, or hematophages. Hb from ingested or parasitized hemoglobin. To better understand the substrate affinity erythrocytes is their major source of exogenous amino acids for Downloaded from of these aspartic proteases, sequences were aligned with growth, development, and reproduction; the Hb, a ϳ64-kDa and/or three-dimensional, molecular models were con- tetrameric polypeptide, is comprehensively catabolized by par- structed of the cathepsin D-like aspartic proteases of asite enzymes to free amino acids or small peptides. Intrigu- schistosomes and hookworms and of plasmepsins of ingly, all these parasites appear to employ cathepsin D-like Plasmodium falciparum and Plasmodium vivax, using aspartic proteases to make initial or early cleavages in the Hb the structure of human cathepsin D bound to the inhib- substrate (2–4).