Structural and Hydrological Influences on the Evolution Of
Total Page:16
File Type:pdf, Size:1020Kb
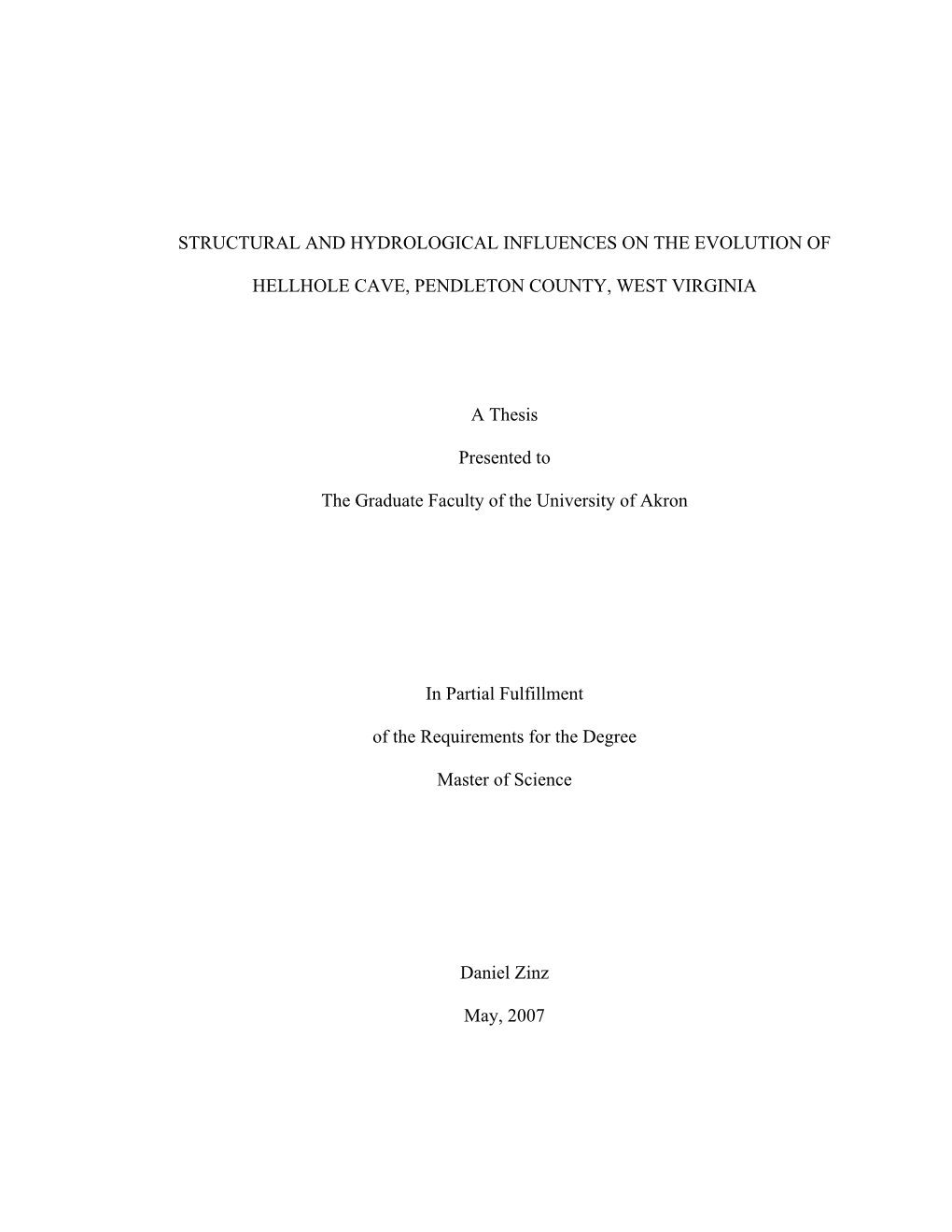
Load more
Recommended publications
-
Area Information
AREA INFORMATION The area is known as the Potomac Highlands. The Allegheny Mountains run right through the region which is the highest watershed for the Potomac River, the largest river feeding into the Chesapeake Bay. The region is renowned for amazing views, high elevation blueberry and spruce stands, dense rhododendron thickets, hundreds of Brook Trout streams, and miles of backcountry trails. Much of the Potomac Highlands is within the one million acre Monongahela National Forest which features National Wilderness areas like Otter Creek, Dolly Sods, Cranberry Glades, Roaring Plains West, and Laurel Fork North. The region is ideal for hiking, rock climbing, skiing, kayaking, canoeing, fishing, and hunting which are all popular activities in the area. The Shavers Fork is a stocked trout stream and maintained by WV Division of Natural Resources, as are many other rivers in the area. Wonderful skiing can be experienced at Timberline, Canaan Valley, Whitegrass Nordic Center Ski areas and Snowshoe Mountain Resort, which are all less than 40 miles from our door. State Parks and forests in the area include Blackwater Falls, Canaan Valley, Audra, Kumbrabow, Seneca, and Cathedral. Federal Recreation areas include Spruce Knob & Seneca Rocks management area, Smoke Hole Canyon, Stuarts Recreation Area, Gaudineer Knob, and Spruce Knob Lake. The region is within 5 hours of half of the nation’s population yet offers a mountain playground second to none. Elkins deserves its high ranking in America's Best Small Art Towns. Elkins is home to Davis and Elkins College and the Augusta Heritage Arts Center, The Mountain State Forest Festival and our thriving Randolph County Community Arts Center (www.randolpharts.org). -
At Base Camp Hanging Around
NEWS OF THE P.A.T.C. MOUNTAINEERING SECTION 1718 N Street, N.W. Washington DC 20036 Vol. 28 No. 4 A r. 1973 Pane mentioned that the climbing area is part of 3000 acres recent- At Base Camp ly donated to a conservation group, and that the blue-blazed trail passing by is PATC maintained. SECTION LAUNCHES WORK TRIP TO SENECA Ed Goodman's Climbers are asked to donate half a day to work on restoring coed explorer post seemed well trained and did a lot of talus slopes at Seneca Rocks, during the weekend May 26-28, in climbing. They had camped there Saturday night. this first area-wide conservation effort undertaken by moun- taineers. John Christian who is spearheading this project in Carderock cooperation with climbers from other clubs urges every MS clim- MD, February 4 Jim Nagy - Trip ber to volunteer in this first work trip and restore through Leader Jay Ozmont Tom Trump common action similar to the Shawangunks undertaking led by June Lehman Bill Bookhout John Stannard what we have destroyed. See climbing calendar, Janet Gladfelter Carol O'Neill page 4, for details. Bob Croft Mark Sollinger Jack Seaber Cliff McGwinn The Milligans Marjorie Coffey Chips Janger ISHI IN TWO WORLDS - Program, Wednesday, May 9 Daniel Weinstein Julie Golden An ecological, anthropological film about the last aboriginal Kevin McCarthy Bob Connor Indian to live in North America who emerged from the hills of Bill Thomas John Birch Northern California in 1908. Marjorie Prochaska John Bonine Tink Peters Mery 8 Fran Oleson He had been alone for 15 years, having outlived the other re- Kevin Miller Chris Scoredos maining members of his tribe with whom he had been isolated in a stone age culture near Mount Lassen since 1870. -
HV September 2009.P65
Volume 42, Number 9 September, 2009 GROUPS PETITION OFFICE OF SURFACE MINING TO TAKE OVER REGULATION OF MINING IN WEST VIRGINIA By John McFerrin The West Virginia Highlands Conservancy, the Sierra Club, Coal mountaintop removal mining. River Mountain Watch, and the Ohio Valley Environmental Coalition have The West Virginia Department of Environmental Protection has petitioned the federal Office of Surface Mining to evaluate the West ignored this rule since its inception. The petition now asks the Office of Virginia State surface mining program, withdraw approval of that pro- Surface Mining to step in and enforce it. gram, and substitute federal enforcement. If successful, the petition The regulation of surface mining is designed to be a partner- would result in the federal Office of Surface Mining (instead of the West ship. In 1977 Congress passed the Surface Mining Control and Recla- Virginia Department of Environmental Protection) regulating mining in mation Act. In that Act, Congress and the federal Office of Surface West Virginia. Mining established minimum standards for the regulation of surface The focus of the petition is the what is known as the buffer zone mining. So long as a state established and enforced equally effective rule. This rule says that a mine cannot disturb the land within one hun- standards it could carry out its own program for the regulation of sur- dred feet of a stream unless certain conditions are met. Such distur- face mining. When it failed to do so, the Office of Surface Mining would bance is prohibited unless the “surface mining activities will not ad- step in and enforce the law. -
NEWS of the P.A.T.C. MOUNTAINEERING SECTION 1718 N Street, N.W., Washington, D.C., 20036
'41 44;41411 Alf ior te4 'Vint° FOUNDED BY JAN AND HERB CONN NEWS OF THE P.A.T.C. MOUNTAINEERING SECTION 1718 N Street, N.W., Washington, D.C., 20036 Vol. XXI No. A ril 1966 CALENDAR OF EVENTS *April 3 Carderock, Maryland April 8-11 Shawangunks, New Paltz, New York LEADER: Larry Griffin (560-8831) Individual parties and conmissaries unless response justifies hiring a bus. Contact leader for information and directions. Alternate trip: Purple Horse, Maryland (Park at Anglers' Inn; cross canal and go upstream to wide- water. Take path on left just before crossing bridge.) April 13 ---- Business Meeting, 1718 N Street, N.W., 8 p.m. PROGRAM: Dick Montague will present a slide lecture on his trip from the Snow Dome high in the Selkirks, hydrographic center of North America, down the Athabasca River. Mr. Montague is a freelance photo-journalist who has traveled the trails of the Hudson's Bay voyageurs for many years, and made this particular tr51; fc.r the Talticnal Geogruhic. April 17 ---- Corkscrew, Blood and Guts, Juliet's Balcony area, Virginia LEADER: Chuck Wettling (843-6217) (Take Beltway to Great Falls, Dranesville exit, where you pick up Route 193 to Great Falls. All cars must be left in Great Falls Park parking area, at a cost of 50 cents. Take main trail downstream to climbing area.) April 24 ---- Little Stogy Man, Virginia LEADER: Ed Goodman (931-2912) (Take Route 211 to Panorama entrance to Skyline Drive, Park in Stony Man parking area, on right hand side of road.) April 25 ---- UP ROPE publication night at 1718 N Street, N.W. -
Monongahela National Forest
Monongahela National Forest Administrative Correction 15 August 12, 2010 Changes to Management Prescription 8.2 Areas Due to New Information Under the 2000 Planning Rule (36 CFR 219.31), the following administrative corrections and additions may be made at any time, are not plan amendments or revisions, and do not require public notice or the preparation of an environmental document under Forest Service NEPA procedures: (1) Corrections and updates of data and maps; (2) Updates to activity lists and schedules as required by § 219.30(d)(1)-(6); (3) Corrections of typographical errors or other non-substantive changes; and (4) Changes in monitoring methods other than those required in a monitoring strategy. The following corrections are a result of new information from the USDI National Park Service about National Natural Landmarks (NNLs) on the Monongahela National Forest (MNF). During a recent visit to inspect NNLs on the MNF, Carolyn Davis of the NPS compared her list of NNLs on the Forest to the Forest Ecologist’s list, and two discrepancies were found. First, the Falls of Hills Creek area was not on the NPS list as an NNL. Second, the Germany Valley Karst Area was on the NPS list but not on the MNF list of NNLs. Therefore, the following administrative corrections are being made to NNLs, which are identified as Management Prescription 8.2 in the MNF Forest Plan. All acreage figures have been rounded off to the nearest 10 acres. CORRECTIONS Forest Plan Chapter III, page III-40 (originally page III-46) MP 8.0 Special Area Table, seventh -
Development of Outdoor Recreation Resource Amenity Indices for West Virginia
Graduate Theses, Dissertations, and Problem Reports 2008 Development of outdoor recreation resource amenity indices for West Virginia Jing Wang West Virginia University Follow this and additional works at: https://researchrepository.wvu.edu/etd Recommended Citation Wang, Jing, "Development of outdoor recreation resource amenity indices for West Virginia" (2008). Graduate Theses, Dissertations, and Problem Reports. 2680. https://researchrepository.wvu.edu/etd/2680 This Thesis is protected by copyright and/or related rights. It has been brought to you by the The Research Repository @ WVU with permission from the rights-holder(s). You are free to use this Thesis in any way that is permitted by the copyright and related rights legislation that applies to your use. For other uses you must obtain permission from the rights-holder(s) directly, unless additional rights are indicated by a Creative Commons license in the record and/ or on the work itself. This Thesis has been accepted for inclusion in WVU Graduate Theses, Dissertations, and Problem Reports collection by an authorized administrator of The Research Repository @ WVU. For more information, please contact [email protected]. Development of Outdoor Recreation Resource Amenity Indices for West Virginia Jing Wang Thesis submitted to the Davis College of Agriculture, Forestry, and Consumer Sciences At West Virginia University in partial fulfillment of the requirements for the degree of Master of Science in Recreation, Parks, and Tourism Resources Jinyang Deng, Ph.D., Chair Chad -
Regional Tectonics
Regional Tectonics Geology 200 Geology for Environmental Scientists Fig.23.10. Differential erosion in horizontal strata Fig. 23.19. Basin & Range model landscape Extension experiment in tectonic modeling lab exercise 2009 Fig. 23.21. Landscape development in basalt plains Tectonics of the Central Appalachians • Appalachian Plateau – Low and High • Valley and Ridge • Great Valley • Blue Ridge High Plateau Great Low Valley Valley Plateau and Ridge Blue Rid ge Relief Map Fig. 23.15. Differential erosion in folded rocks Relief Map with county boundaries Satellite Image Variation in fold wave length vs. fold amplitude. Open folds are typical of the Appalachian Plateau , whereas tight folds are typical of the Valley and Ridge. Isoclinal folds are not common in the Appalachians. View of open folds on the Appalachian Plateau, Garrett Co., Maryland. West-dipping Hogbacks Geologic Cross Section from Seneca Rocks to Elkins, WV Thrustinggpp of the Wills Mtn Duplex created open folds to the west. Plateau Seneca Rocks Valley & Ridge Geologic Map of the central Appalachians (Dunne, 1996) Various interpretations of Valley and Ridge geology (Dunne, 1996) WMA= Wills Mtn. Anticline, BTS= Broad Top Synclinorium, CMA= Cacapon Mtn. An tic line, NMT = N or th Mt n. Thrust Cross section by Kulander and Dean (1986) Tuscarora Ss. ifin roof sequence Cambro-Ordovician carbonates Compression experiment in tectonic modlideling l lbab exerci se 2009 2009 Preferred cross section of Dunne (1996) Roof sequence Thrust sheets Deformed quartz vein approximately 100 m long. AlllA small scale exampl lfflddhble of a fold and thrust belt. Folded soil layer along a lake in Wisconsin caused by thrusting of ice on shore byyg high winds durin gpgg spring thaw. -
Early Results from Calcium Carbonate Neutralization of Two West Virginia Rivers Acidified by Mine Drainage
EARLY RESULTS FROM CALCIUM CARBONATE NEUTRALIZATION OF TWO WEST VIRGINIA RIVERS ACIDIFIED BY MINE DRAINAGE Peter E. Zurbuch West Virginia Division of Natural Resources Elkins, WV Presented at the Seventeenth Annual West Virginia Surface Mine Drainage Task Force Symposium, Morgantown, WV, April 2‐3, 1996. Introduction In 1992 the State of West Virginia initiated a stream restoration program directed to waters affected by acid mine drainage (AMD). The West Virginia Division of Environmental Protection (WV DEP) was placed in charge of the program. A technical committee, consisting of representatives from state and federal agencies and the private sector, was charged with developing data on priority streams and recommending methods for their restoration. The West Virginia Division of Natural Resources (WV DNR) has members on the committee because of their experience treating streams acidified by acid deposition. The first two streams selected for restoration are being treated using methods developed by the WV DNR and successfully applied to streams acidified by acid deposition. This paper briefly reviews these methods and their application to the Blackwater River in Tucker County and the Middle Fork River in Randolph, Upshur and Barbour counties. Background The WV DNR began studying methods of treating lightly buffered acid streams in the late 1950's. The early history of this work has been reviewed previously by this author (Zurbuch 1984). One of the first methods tried was the placement of 2‐inch diameter limestone aggregate in the bed of a small stream. The method was abandoned when after a short period of time the stone became coated with precipitates and non‐reactive. -
North Fork Mountain and Thorn Creek CFA Action Plan
Action Plan for the North Fork Mountain & Thorn Creek Conservation Focus Area West Virginia Division of Natural Resources January 2021 Table of Contents Executive Summary ........................................................................................................................................... 1 Introduction to the State Wildlife Action Plan & Conservation Focus Areas .................................................... 2 Species of Greatest Conservation Need, Habitats and Stresses ................................................................... 2 Conservation Actions .................................................................................................................................... 2 Conservation Focus Areas and Action Plans ................................................................................................. 3 Climate Change and Resilience ..................................................................................................................... 4 Monitoring and Adaptive Management ....................................................................................................... 5 Organization of this Action Plan .................................................................................................................... 6 How to use this plan ..................................................................................................................................... 7 North Fork Mountain and Thorn Creek Conservation Focus Area .................................................................. -
Examiner Moorefield
AWARDED for GENERAL EXCELLENCE in ADVERTISING — 2009, 2010, 2011, 2012, 2014, 2015 & in EDITORIAL—2008, 2009, 2010, 2011, 2012, 2015 Heritage Weekend Brightens Page 8B Otherwise Dreary Weekend #2 Cougars Clear Tygarts Valley | #3 Yellow Jackets Cash in on Antoher Summit Bowl Victory Page 1B Established 1845 Wednesday, September 30, 2015 OOREFIELD XAMINER MVOLUME 124 - NUMBER 39 TWO SECTIONS • 16 PAGES 94¢ E USPS 362-300 and Hardy County News OSHA Says Amputation, BOE Sees 2014- Other Injuries at Pilgrim’s 2015 Test Scores By Jean A. Flanagan testing, according to data present- Moorefield Examiner ed by Director of Elementary Cur- riculum Beverly Coppe. Hardy County students had Coppe presented information to Pride Were ‘Preventable’ mixed results with the first year of the Hardy County Board of Edu- the Smarter Balance proficiency Continued on page 7 In less than 60 days, three employee inju- St. and 129 Potomac Ave. in Moorefield. As access. ries – including one worker who suffered the a result, OSHA cited the company on Sept. A third worker had three fingers amputated amputation of three fingers – brought federal 11 for one repeat and three serious violations. when they caught in a blender while removing inspectors to West Virginia poultry processing Proposed fines total $46,825. poultry from the machine on June 15. Smarter Balance Scores facilities operated by Pilgrim’s Pride Corp., On April 28, 2015, an employee contacted “Amputations have lasting professional and one of the world’s largest chicken producers. an unguarded machine shaft while operating a personal consequences – and these injuries 2014-15 School Year U.S. -
09 September 2017
highlands-50-cover.pdf 1 9/1/17 11:04 AM The Highlands Voice September, 2017 Page 2 Ramblin’ the Ridges By Cynthia D. Ellis Steady gaze Welcome! Greetings! Salutations! waters fouled by those same spoils and business.” He meant that they would like Whether you are with us in person at our mine drainages too. We could envisage to lay aside the business of patching up th 50 anniversary celebration, or checking up precious public lands whittled away or injured birds, because ideally there would on us by reading this at home, we send you commercialized beyond recognition. no birds suffering injuries, especially those our very best wishes for being part of the Alternately, we could take an upbeat that are related to humans. first 50 years of the West Virginia Highlands tack. We could foresee years ahead with In the future, is that what we Conservancy. folks joining our group and ally groups wish? Do we hope there will be no West Throughout this year we’ve looked and new groups to make change for better Virginia Highlands Conservancy, because back at our past. But now, what’s the highlands will be in a state of next? protection and preservation? Do we Periodically writers for The want to be out of a “job”? Highlands Voice have toyed with No. But maybe our work will forecasting the future. be different and the burden will be “On July 4, 1972, hundreds even more widely shared. of visitors turned away disappointed In 1973, in our 8th year, noted during the long holiday weekend Charleston Daily Mail columnist when smog prevented the sightseers Skip Johnson wrote a piece from catching a glimpse of the complimenting us and said, “Every spectacular falls.” That’s how one of government bureaucracy, every coal us, in 1969, fancied that Blackwater executive, every politician needs a Falls could be affected by the air suspicious citizen looking over his pollution problems then. -
Workshop Agenda and Notes
Spruce Knob-Seneca Rocks National Recreation Area Climbing Management Plan Workshop 1D – September 1, 2020 Notes and Agenda Agenda 6:00-6:25 Alex Schlueter introduction and presentation 6:25-6:35 Questions on presentation/process 6:35-7:40 Small group discussions (self-introductions and follow questions) 7:40-8:00 Report out from each group 6:35-7:40 Small group discussions There were approximately 12 participants so it was not necessary to split into smaller groups. The following pages are the notes guided by discussion questions. Spruce Knob-Seneca Rocks National Recreation Area Climbing Management Plan Workshop 1D – September 1, 2020 Notes 1. Self-Introductions of Group Leader and participants 2. Code of conduct and technology tips Be courteous and respectful of other people's opinions: failure to adhere to this, or other inappropriate behavior, may result in removal from the meeting Be understanding of the limitations of the technology and exercise patience if and when technical problems arise Mute your microphone when not speaking; non-verbal communication is encouraged (nodding; thumbs up, hand-raising etc.) Be mindful of lag in audio and consider allowing some space between speaking Be mindful that you are on camera and try to avoid things that could cause a distraction 3. What do you value about the area or crag(s) that you climb in this area? Please be specific as to which places you are associating your response with. (15 min) Further leading questions: - What motivates you to select that crag? Is it the setting, the social