Laser Calorimetry Spectroscopy for Ppm-Level Dissolved Gas Detection and Analysis Received: 01 September 2016 Nagapriya K
Total Page:16
File Type:pdf, Size:1020Kb
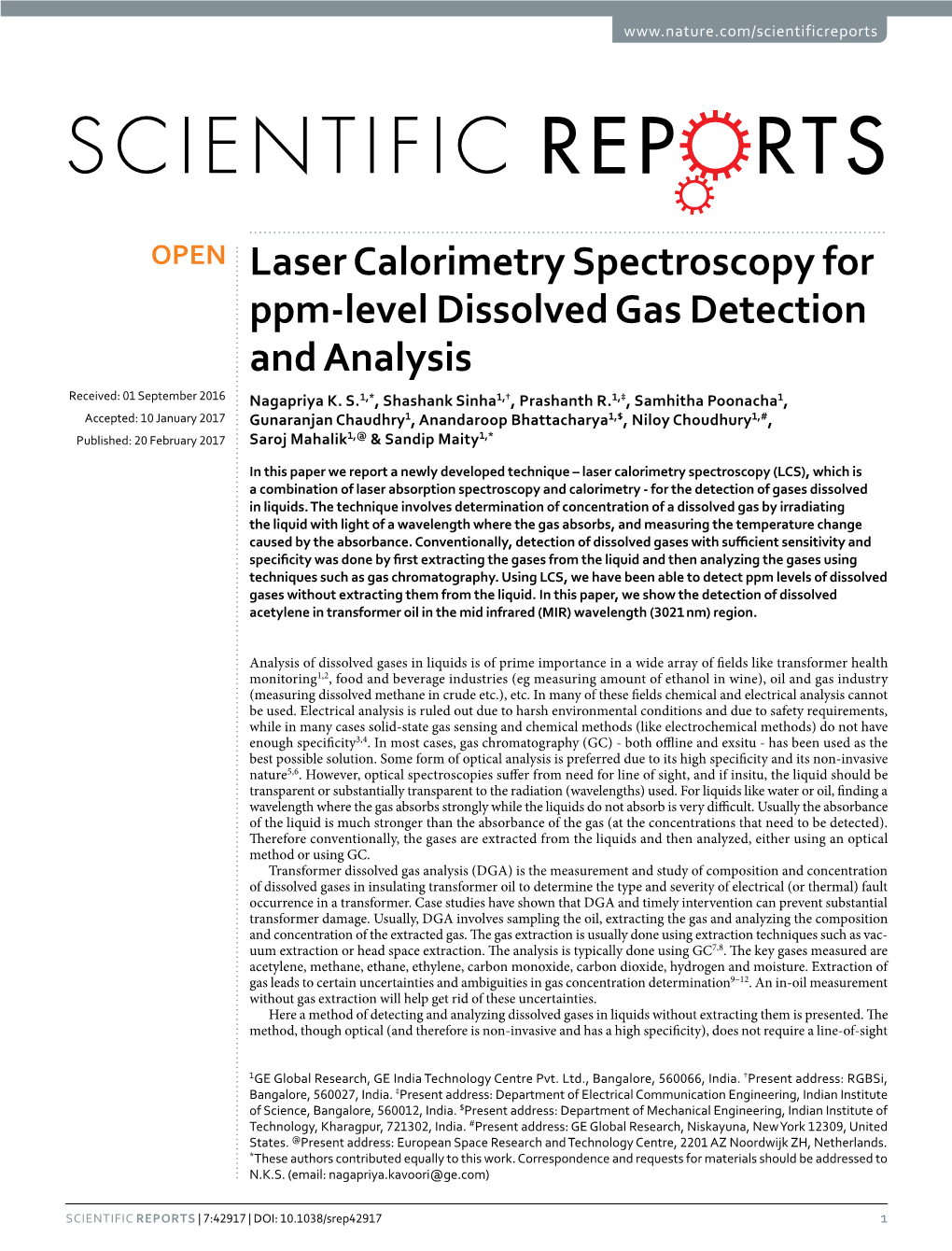
Load more
Recommended publications
-
Chemistry Grade Level 10 Units 1-15
COPPELL ISD SUBJECT YEAR AT A GLANCE GRADE HEMISTRY UNITS C LEVEL 1-15 10 Program Transfer Goals ● Ask questions, recognize and define problems, and propose solutions. ● Safely and ethically collect, analyze, and evaluate appropriate data. ● Utilize, create, and analyze models to understand the world. ● Make valid claims and informed decisions based on scientific evidence. ● Effectively communicate scientific reasoning to a target audience. PACING 1st 9 Weeks 2nd 9 Weeks 3rd 9 Weeks 4th 9 Weeks Unit 1 Unit 2 Unit 3 Unit 4 Unit 5 Unit 6 Unit Unit Unit Unit Unit Unit Unit Unit Unit 7 8 9 10 11 12 13 14 15 1.5 wks 2 wks 1.5 wks 2 wks 3 wks 5.5 wks 1.5 2 2.5 2 wks 2 2 2 wks 1.5 1.5 wks wks wks wks wks wks wks Assurances for a Guaranteed and Viable Curriculum Adherence to this scope and sequence affords every member of the learning community clarity on the knowledge and skills on which each learner should demonstrate proficiency. In order to deliver a guaranteed and viable curriculum, our team commits to and ensures the following understandings: Shared Accountability: Responding -
Calorimetry the Science of Measuring Heat Generated Or Consumed During a Chemical Reaction Or Phase Change
February 05, 2014 Calorimetry the science of measuring heat generated or consumed during a chemical reaction or phase change What is going to happen when the burner is lit? February 05, 2014 Experimental Design Chemical changes involve potential energy, which cannot be measured directly Chemical reactions absorb or release energy with the surroundings, which cause a change in temperature of the surroundings (and this can be measured) In a calorimetry experiment, we set up the experiment so all of the energy from the reaction (the system) is exchanged with the surroundings ∆Esystem = ∆Esurroundings Chemical System Surroundings (water) Endothermic Change February 05, 2014 Chemical System Surroundings (water) Exothermic Change ∆Esystem = ∆Esurroundings February 05, 2014 There are three main calorimeter designs: 1) Flame calorimeter -a fuel is burned below a metal container filled with water 2) Bomb Calorimeter -a reaction takes place inside an enclosed vessel with a surrounding sleeve filled with water 3) Simple Calorimeter -a reaction takes place in a polystyrene cup filled with water Example #1 A student assembles a flame calorimeter by putting 350 grams of water into a 15.0 g aluminum can. A burner containing ethanol is lit, and as the ethanol is burning the temperature of the water increases by 6.30 ˚C and the mass of the ethanol burner decreases by 0.35 grams. Use this information to calculate the molar enthalpy of combustion of ethanol. February 05, 2014 The following apparatus can be used to determine the molar enthalpy of combustion of butan-2-ol. Initial mass of the burner: 223.50 g Final mass of the burner : 221.25 g water Mass of copper can + water: 230.45 g Mass of copper can : 45.60 g Final temperature of water: 67.0˚C Intial temperature of water : 41.3˚C Determine the molar enthalpy of combustion of butan-2-ol. -
"Thermal Analysis and Calorimetry," In
Article No : b06_001 Thermal Analysis and Calorimetry STEPHEN B. WARRINGTON, Formerly Anasys, IPTME, Loughborough University, Loughborough, United Kingdom Gu€NTHER W. H. Ho€HNE, Formerly Polymer Technology (SKT), Eindhoven University of Technology, Eindhoven, The Netherlands 1. Thermal Analysis.................. 415 2.2. Methods of Calorimetry............. 424 1.1. General Introduction ............... 415 2.2.1. Compensation of the Thermal Effects.... 425 1.1.1. Definitions . ...................... 415 2.2.2. Measurement of a Temperature Difference 425 1.1.2. Sources of Information . ............. 416 2.2.3. Temperature Modulation ............. 426 1.2. Thermogravimetry................. 416 2.3. Calorimeters ..................... 427 1.2.1. Introduction ...................... 416 2.3.1. Static Calorimeters ................. 427 1.2.2. Instrumentation . .................. 416 2.3.1.1. Isothermal Calorimeters . ............. 427 1.2.3. Factors Affecting a TG Curve ......... 417 2.3.1.2. Isoperibolic Calorimeters ............. 428 1.2.4. Applications ...................... 417 2.3.1.3. Adiabatic Calorimeters . ............. 430 1.3. Differential Thermal Analysis and 2.3.2. Scanning Calorimeters . ............. 430 Differential Scanning Calorimetry..... 418 2.3.2.1. Differential-Temperature Scanning 1.3.1. Introduction ...................... 418 Calorimeters ...................... 431 2.3.2.2. Power-Compensated Scanning Calorimeters 432 1.3.2. Instrumentation . .................. 419 2.3.2.3. Temperature-Modulated 1.3.3. Applications ...................... 419 Scanning Calorimeters . ............. 432 1.3.4. Modulated-Temperature DSC (MT-DSC) . 421 2.3.3. Chip-Calorimeters .................. 433 1.4. Simultaneous Techniques............ 421 2.4. Applications of Calorimetry.......... 433 1.4.1. Introduction ...................... 421 2.4.1. Determination of Thermodynamic Functions 433 1.4.2. Applications ...................... 421 2.4.2. Determination of Heats of Mixing . .... 434 1.5. Evolved Gas Analysis.............. -
THERMOCHEMISTRY – 2 CALORIMETRY and HEATS of REACTION Dr
THERMOCHEMISTRY – 2 CALORIMETRY AND HEATS OF REACTION Dr. Sapna Gupta HEAT CAPACITY • Heat capacity is the amount of heat needed to raise the temperature of the sample of substance by one degree Celsius or Kelvin. q = CDt • Molar heat capacity: heat capacity of one mole of substance. • Specific Heat Capacity: Quantity of heat needed to raise the temperature of one gram of substance by one degree Celsius (or one Kelvin) at constant pressure. q = m s Dt (final-initial) • Measured using a calorimeter – it absorbed heat evolved or absorbed. Dr. Sapna Gupta/Thermochemistry-2-Calorimetry 2 EXAMPLES OF SP. HEAT CAPACITY The higher the number the higher the energy required to raise the temp. Dr. Sapna Gupta/Thermochemistry-2-Calorimetry 3 CALORIMETRY: EXAMPLE - 1 Example: A piece of zinc weighing 35.8 g was heated from 20.00°C to 28.00°C. How much heat was required? The specific heat of zinc is 0.388 J/(g°C). Solution m = 35.8 g s = 0.388 J/(g°C) Dt = 28.00°C – 20.00°C = 8.00°C q = m s Dt 0.388 J q 35.8 g 8.00C = 111J gC Dr. Sapna Gupta/Thermochemistry-2-Calorimetry 4 CALORIMETRY: EXAMPLE - 2 Example: Nitromethane, CH3NO2, an organic solvent burns in oxygen according to the following reaction: 3 3 1 CH3NO2(g) + /4O2(g) CO2(g) + /2H2O(l) + /2N2(g) You place 1.724 g of nitromethane in a calorimeter with oxygen and ignite it. The temperature of the calorimeter increases from 22.23°C to 28.81°C. -
Isothermal Titration Calorimetry and Differential Scanning Calorimetry As Complementary Tools to Investigate the Energetics of Biomolecular Recognition
JOURNAL OF MOLECULAR RECOGNITION J. Mol. Recognit. 1999;12:3–18 Review Isothermal titration calorimetry and differential scanning calorimetry as complementary tools to investigate the energetics of biomolecular recognition Ilian Jelesarov* and Hans Rudolf Bosshard Department of Biochemistry, University of Zurich, CH-8057 Zurich, Switzerland The principles of isothermal titration calorimetry (ITC) and differential scanning calorimetry (DSC) are reviewed together with the basic thermodynamic formalism on which the two techniques are based. Although ITC is particularly suitable to follow the energetics of an association reaction between biomolecules, the combination of ITC and DSC provides a more comprehensive description of the thermodynamics of an associating system. The reason is that the parameters DG, DH, DS, and DCp obtained from ITC are global properties of the system under study. They may be composed to varying degrees of contributions from the binding reaction proper, from conformational changes of the component molecules during association, and from changes in molecule/solvent interactions and in the state of protonation. Copyright # 1999 John Wiley & Sons, Ltd. Keywords: isothermal titration calorimetry; differential scanning calorimetry Received 1 June 1998; accepted 15 June 1998 Introduction ways to rationalize structure in terms of energetics, a task that still is enormously difficult inspite of some very Specific binding is fundamental to the molecular organiza- promising theoretical developments and of the steady tion of living matter. Virtually all biological phenomena accumulation of experimental results. Theoretical concepts depend in one way or another on molecular recognition, have developed in the tradition of physical-organic which either is intermolecular as in ligand binding to a chemistry. -
Calorimetry Has Been Routinely Used at US and European Facilities For
10. PRINCIPLES AND APPLICATIONS OF CALORIMETRIC ASSAY D. S. Bracken, and C. R. Rudy I. Introduction Calorimetry is the quantitative measurement of heat. Applications of calorimetry include measurements of the specific heats of elements and compounds, phase-change enthalpies, and the rate of heat generation from radionuclides. The most successful radiometric calorimeter designs fit the general category of heat-flow calorimeters. Calorimetry is used as a nondestructive assay (NDA) technique for determining the power output of heat-producing nuclear materials. The heat is generated by the decay of radioactive isotopes within the item. Because the heat-measurement result is completely independent of material and matrix type, it can be used on any material form or item matrix. Heat-flow calorimeters have been used to measure thermal powers from 0.5 mW (0.2 g low-burnup plutonium equivalent) to 1,000 W for items ranging in size from less than 2.54 cm to 60 cm in diameter and up to 100 cm in length. Calorimetric assay is the determination of the mass of radioactive material through the combined measurement of its thermal power by calorimetry and its isotopic composition by gamma-ray spectroscopy or mass spectroscopy. Calorimetric assay has been routinely used at U.S. and European facilities for plutonium process measurements and nuclear material accountability for the last 40 years [EI54, GU64, GU70, ANN15.22, AS1458, MA82, IAEA87]. Calorimetric assay is routinely used as a reliable NDA technique for the quantification of plutonium and tritium content. Calorimetric assay of tritium and plutonium-bearing items routinely obtains the highest precision and accuracy of all NDA techniques. -
Calorimetry - 2018
Physikalisch-chemisches Praktikum I Calorimetry - 2018 Calorimetry Summary Calorimetry can be used to determine the heat flow in chemical reactions, to mea- sure heats of solvation, characterize phase transitions and ligand binding to pro- teins. Theoretical predictions of bond energies or of the stability and structure of compounds can be tested. In this experiment you will become familiar with the basic principles of calorimetry. You will use a bomb calorimeter, in which chemical reactions take place without change of volume (isochoric reaction path). The tem- perature change in a surrounding bath is monitored. You will compute standard enthalpies of formation and perform a thorough error analysis. Contents 1 Introduction2 1.1 Measuring heat.................................2 1.2 Calorimetry Today...............................2 2 Theory3 2.1 Heat transfer in different thermodynamic processes.............3 2.2 Temperature dependence and heat capacity.................5 2.3 Different Reaction paths and the law of Hess.................6 3 Experiment8 3.1 Safety Precautions...............................8 3.2 Experimental procedure............................8 3.3 Thermometer Calibration........................... 11 4 Data analysis 11 4.1 Determination of the temperature change ∆T ................ 11 4.2 Calibration of the calorimeter......................... 11 4.3 Total heat of combustion at standard temperature.............. 13 4.4 Correction for the ignition wire........................ 13 4.5 Nitric acid correction.............................. 13 4.6 Standard heat of combustion of the sample.................. 14 4.7 Reaction Enthalpy and Enthalpy of Formation................ 14 5 To do List and Reporting 14 A Error analysis for the heat capacity of the calorimeter 16 B Error analysis for ∆qTs of the sample 17 C Fundamental constants and useful data[1] 18 Page 1 of 19 Physikalisch-chemisches Praktikum I Calorimetry - 2018 1 Introduction 1.1 Measuring heat Heat can not be measured directly. -
TAM AIR ISOTHERMAL CALORIMETRY High Sensitivity
TAM AIR ISOTHERMAL CALORIMETRY High Sensitivity Multi-Sample TAM AIR The best choice Long-Term 3 or 8 for isothermal Temperature Channel calorimetry of Stability Option chemical reactions and metabolic processes The TAM Air isothermal calorimetry system provides the sample flexibility and measurement sensitivity to accurately characterize heat flow in a wide variety of materials. The 8-channel Lowest calorimeters are widely recognized as the instrument of choice Baseline for cement hydration analyses using ASTM C1702 and C1679. The 3-channel calorimeters have the required sensitivity to detect Drift low levels of metabolic activity required for applications such as soil remediation and food contamination analysis. Unmatched baseline stability and signal-to-noise performance makes the TAM Air the best choice for the analysis of medium to high heat- producing materials. TAM AIR A VERSATILE ISOTHERMAL CALORIMETER with HIGH PERFORMANCE & ROBUSTNESS The TAM Air is a flexible, sensitive analytical platform that is an ideal tool for large-scale isothermal calorimetry experiments. Capable of measuring several samples simultaneously under isothermal conditions, the TAM Air with its air-based thermostat and easily interchangeable 8-channel standard volume or 3-channel large volume calorimeters, is especially well-suited for characterizing processes that evolve or consume heat over the course of days and weeks. The TAM Air offers high sensitivity and long-term temperature stability for detecting and characterizing heat production or consumption in a wide variety of sample types and sizes. Applications include cement and concrete hydration, food spoilage, microbial activity, battery performance and more. Monitoring the thermal activity or heat flow of chemical, physical and biological processes provides information which cannot be generated with other techniques. -
Chapter 5 Thermochemistry Learning Objectives ______
AY2016-2017 Chapter 5 Thermochemistry Learning Objectives _____________________________________________________________________________________ To satisfy the minimum requirements for this course, you should be able to: 1. Discuss the nature of energy by: • comparing and contrasting kinetic and potential energy • identifying heat (q) and work (w) as the two forms of transient energy (energy transfer) • distinguishing between heat (q), internal energy (U) and temperature (T) 2. Demonstrate an understanding of thermochemistry by: • explaining the relationships among the following: system, surroundings, and universe; exothermic process and endothermic process; internal energy (U) and enthalpy (H); ∆U, ∆H, qv, and qp • associating the sign of ∆H with whether the process is exothermic or endothermic • calculating the quantity of heat involved in a reaction given the quantity of reactants and the enthalpy change for the reaction • calculating the amount of reactant needed to generate a given amount of heat • distinguishing between state functions and path functions and identifying examples of each • stating the first law of thermodynamics in words and performing calculations using the first law for a closed system (∆U = q + w) • explaining the sign conventions for heat and work 3. Demonstrate an understanding of the concept of calorimetry by: • understanding the concept of specific heat capacity (C) • performing calculations using the equation q = C∆T (or q = mass Cs ΔT, q = mol Cm ΔT) • using constant pressure calorimetry data to calculate the standard reaction enthalpy (∆rHº = qp) or to calculate the specific heat of a substance 4. Name the six phase change processes and be able to: • use Kinetic Molecular Theory to explain the relative energy changes associated with each phase change (∆Hvap, ∆Hfus, ∆Hsub) • use heat capacities and heats of fusion and vaporization to calculate the heat absorbed or evolved when a substance is heated or cooled and undergoes phase changes 5. -
Application of Scanning Calorimetry to Estimate Soil Organic Matter Loss After fires
J Therm Anal Calorim (2011) 104:351–356 DOI 10.1007/s10973-010-1046-8 Application of scanning calorimetry to estimate soil organic matter loss after fires Sergey V. Ushakov • Divya Nag • Alexandra Navrotsky Received: 10 July 2010 / Accepted: 7 September 2010 / Published online: 21 September 2010 Ó The Author(s) 2010. This article is published with open access at Springerlink.com Abstract The severe heating of soil during wildfires and (SOC) concentration dates back to 1900 [1] and relies on prescribed burns may result in adverse effects on soil fer- quantitative analysis of CO2 evolved on combustion of soil tility due to organic matter loss. No rapid and reliable organic matter. Today this procedure is considered the procedure exists to evaluate soil organic matter (SOM) most accurate, but the high cost of modern dry combustion losses due to heating. Enthalpy of SOM combustion cor- instruments is a limitation to many laboratories [2]. Acid relates with organic matter content. Quartz is a ubiquitous pretreatment is often required for correction for inorganic mineral in soils and has a remarkably constant composition carbon from carbonate minerals present in soil samples. and reversible a–b phase transition at 575 °C. We suggest A wet chemical oxidation method (sometimes referred to that SOM content in heated and unheated soils can be as ‘‘wet combustion’’) was suggested in 1934 as a ‘‘proce- compared using the ratio of SOM combustion enthalpy on dure more rapid than others so far proposed useful for heating to the b–a quartz transition enthalpy measured on comparative purposes where no very exact determination is cooling of the same sample. -
Electrochemical Microcalorimetry
Electrochemical Microcalorimetry Kai Etzel, Katrin Bickel and Rolf Schuster Physical Chemistry, Karlsruhe Institute of Technology, Germany research interests: -Surfaces in vacuum and electrochemical environment structure, phase transition, ordering processes 10 nm ‚electronic structure‘, scanning tunneling spectroscopy (electrochemical STM, XPS, …) -Electrochemical microstructuring 0 -Thermodynamics and kinetics of electrochemical reactions metal deposition, H-adsorption/evolution (electrochemical STM, [mK] Temperature -0.3 0 0.1 0.2 microcalorimetry, surface plasmon resonance,…) Time [s] 1 Rolf Schuster Institute of Physical Chemistry, Physical Chemistry of Condensed Matter Electrochemical Microcalorimetry Kai Etzel, Katrin Bickel and Rolf Schuster Physical Chemistry, Karlsruhe Institute of Technology, Germany Historical: E. J. Mills, „On Electrostriction“, Proc. Roy. Soc. Lond. 26, 504 (1877) E. Bouty, „Sur un phénomène analogue au phénomène de Peltier“, Comptes Rendus 89, 146 (1879) Cu-deposition Cu-dissolution ⇒ decreasing temperature ⇒ increasing temperature „electrochemical Peltier heats“ 2- SO4 Cu2+ Cu-plated 2 Rolf Schuster Institute of Physical Chemistry, Physical Chemistry of Condensed Matter What do we learn from electrochemical microcalorimetry? In „conventional“ calorimetry: qm = Δ R H; p,T = const. In electrochemical calorimetry: electrical work: wel,m = z ⋅ F ⋅φ = −Δ RG = −(Δ R H −TΔ R S); from the ‚chemical reaction‘ heat transfer from surrounding ⇒ qm = Δ RG − Δ R H = −TΔ R S; Ostwald (1903) We measure the reaction entropy, -
The Development of Stirred-Tank Heat Flow Calorimetry As a Tool For
SAFETY AND ENVIRONMENTAL PROTECTION IN CHEMISTRY 189 CHIMIA 5/ (1997) Nr. 5 (Mail Chimia 51 (1997) 189-200 drugs, crop-protection agents, coat-pro- © Neue SchlVeizerische Chemische Gesellschaft tecting additives, dyes or pigments) in a ISSN 0009-4293 form (or formulation) which the consumer needs or particularly likes. It must be eco- nomic but also safe and ecologically ac- The Development of Stirred- ceptable. The task of chemical process develop- Tank Heat Flow Calorimetry as ment is to elaborate such processes effec- tively and efficiently. Fast implementa- a Tool for Process Optimization tion of new processes is becoming in- creasingly important. Ideally, in a well- and Process Safetya) developed process, we have a basic under- standing of all reaction steps (kinetics, influence of process parameters on selec- Willy Regenass* tivity, potential of thermally hazardous conditions) and a quantitative, model- based understanding of separation stages. Abstract. Calorimetry based on the measurement of heat release rates has found The tools of process development have widespread use in chemical process work, particularly for aspects of thermal process improved in recent decades to an impres- hazards. However, it has not yet found the breadth of application it deserves. This sive extent. The most important of these contribution discusses the tasks and problems of chemical process development and the tools are: role of heat flow calorimetry in this context. It reviews the historical development of - methods of analysis to follow the com- heat flow calorimetry and analyzes the prerequisites for a successful development of the position of reaction mixtures and method in the future. streams in separation processes, - methods for physical property estima- tion, 1.