Crosstalk Between Proteins Expression and Lysine Acetylation In
Total Page:16
File Type:pdf, Size:1020Kb
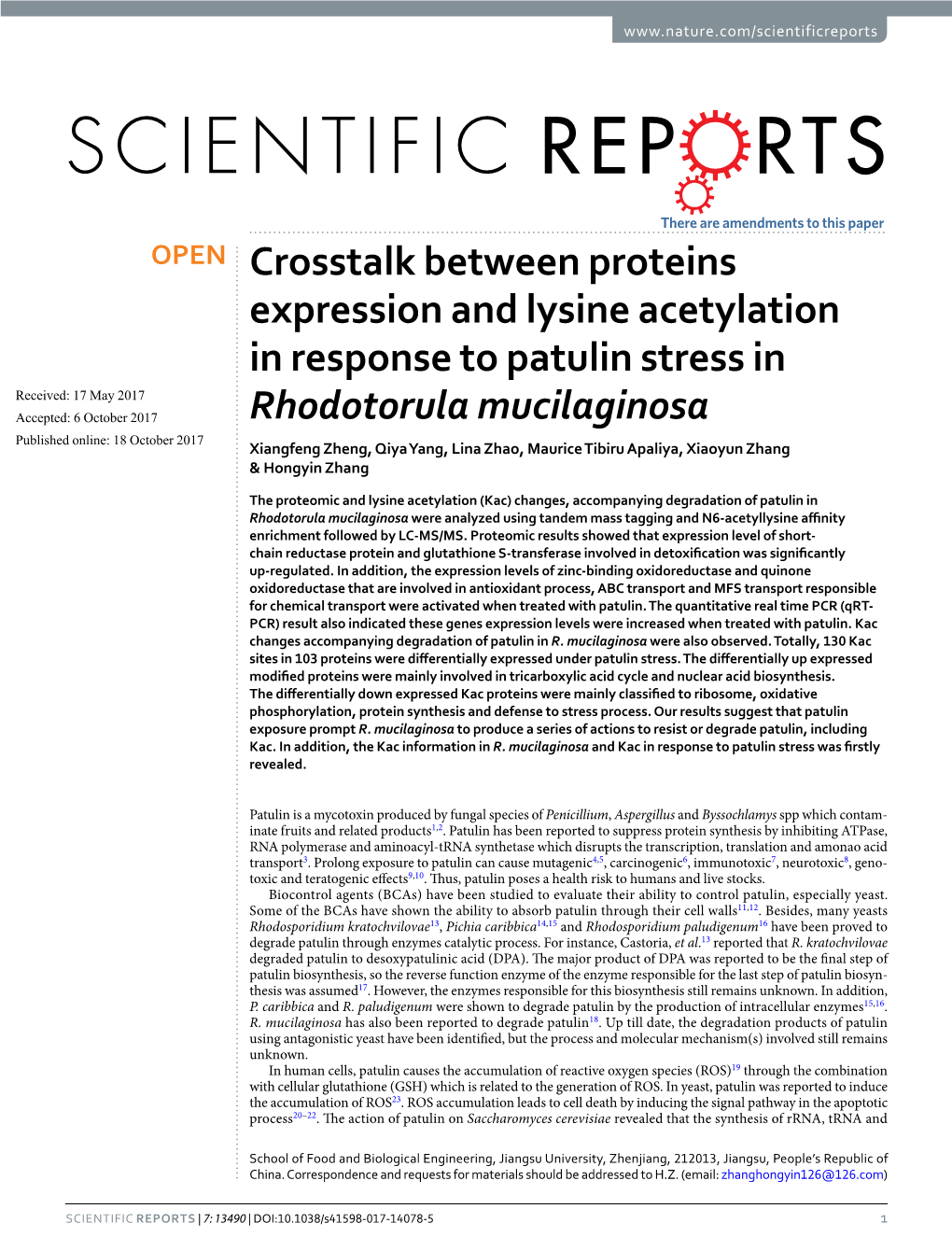
Load more
Recommended publications
-
Investigating the Biological Role of O-Acyl ADP Ribose
Investigating the Biological Role of O-Acyl ADP Ribose by Elyse Blazosky A thesis submitted to Johns Hopkins University in conformity with the requirements for the degree of Master of Science Baltimore, Maryland November, 2018 © Elyse Blazosky All Rights Reserved Abstract Sirtuins are an ancient family of deacetylase enzymes found in all three domains of life, where they have diverse biological roles. These widely studied enzymes are popular drug targets for treating diseases associated with aging, neurological disorders, cardiovascular disorders, metabolic disorders and even cancer. Unlike most deacetylase enzymes which use water to hydrolyze the amide bond linking the acetyl group to a lysine side chain, sirtuins catalyze a unique NAD+-dependent reaction that yields O-acetyl ADP ribose, nicotinamide and the deacetylate lysine. This seemingly wasteful use of NAD+ has led some to hypothesize that sirtuin activity is coupled to NAD+ levels in the cell. While sirtuin activity does rely on NAD+ biosynthesis and salvage pathways, it is unclear whether NAD+ levels fluctuate to a level that could affect sirtuin activity in-vivo. More recent studies have revealed new roles for sirtuins which suggests a more complex role of the sirtuin and a re-evaluation of the current hypothesis for why sirtuins uses NAD+. It has been shown that some sirtuins preferentially remove a variety of acyl lysine groups such as malonyl, succinyl, and butyryl, forming the corresponding O-acyl ADP ribose product. Mass spectrometry studies have revealed an abundance of these acyl modifications on cellular proteins, some of which are thought to result from non-enzymatic reaction with metabolites such as acyl-CoAs. -
Computational Modeling of Lysine Post-Translational Modification: an Overview Md
c and S eti ys h te nt m y s S B Hasan MM et al., Curr Synthetic Sys Biol 2018, 6:1 t i n o e l Current Synthetic and o r r g DOI: 10.4172/2332-0737.1000137 u y C ISSN: 2332-0737 Systems Biology CommentaryResearch Article OpenOpen Access Access Computational Modeling of Lysine Post-Translational Modification: An Overview Md. Mehedi Hasan 1*, Mst. Shamima Khatun2, and Hiroyuki Kurata1,3 1Department of Bioscience and Bioinformatics, Kyushu Institute of Technology, 680-4 Kawazu, Iizuka, Fukuoka 820-8502, Japan 2Department of Statistics, Laboratory of Bioinformatics, Rajshahi University-6205, Bangladesh 3Biomedical Informatics R&D Center, Kyushu Institute of Technology, 680-4 Kawazu, Iizuka, Fukuoka 820-8502, Japan Commentary hot spot for PTMs, and a number of protein lysine modifications could occur in both histone and non-histone proteins [11,12]. For instance, Living organisms have a magnificent ordered and complex lysine methylation in non-histone proteins can regulate the protein structure. In regulating the cellular functions, post-translational activity and protein structure stability [13]. In 2004, the Nobel Prize in modifications (PTMs) are critical molecular measures. They alter Chemistry was awarded jointly to Aaron Ciechanover, Avram Hershko protein conformation, modulating their activity, stability and and Irwin Rose for the discovery of lysine ubiquitin-mediated protein localization. Up to date, more than 300 types of PTMs are experimentally degradation [14]. discovered in vivo and in vitro pathways [1,2]. Major and common PTMs are methylation, ubiquitination, succinylation, phosphorylation, Moreover, in biological process, lysine can be modified by the glycosylation, acetylation, and sumoylation. -
SUMO and Transcriptional Regulation: the Lessons of Large-Scale Proteomic, Modifomic and Genomic Studies
molecules Review SUMO and Transcriptional Regulation: The Lessons of Large-Scale Proteomic, Modifomic and Genomic Studies Mathias Boulanger 1,2 , Mehuli Chakraborty 1,2, Denis Tempé 1,2, Marc Piechaczyk 1,2,* and Guillaume Bossis 1,2,* 1 Institut de Génétique Moléculaire de Montpellier (IGMM), University of Montpellier, CNRS, Montpellier, France; [email protected] (M.B.); [email protected] (M.C.); [email protected] (D.T.) 2 Equipe Labellisée Ligue Contre le Cancer, Paris, France * Correspondence: [email protected] (M.P.); [email protected] (G.B.) Abstract: One major role of the eukaryotic peptidic post-translational modifier SUMO in the cell is transcriptional control. This occurs via modification of virtually all classes of transcriptional actors, which include transcription factors, transcriptional coregulators, diverse chromatin components, as well as Pol I-, Pol II- and Pol III transcriptional machineries and their regulators. For many years, the role of SUMOylation has essentially been studied on individual proteins, or small groups of proteins, principally dealing with Pol II-mediated transcription. This provided only a fragmentary view of how SUMOylation controls transcription. The recent advent of large-scale proteomic, modifomic and genomic studies has however considerably refined our perception of the part played by SUMO in gene expression control. We review here these developments and the new concepts they are at the origin of, together with the limitations of our knowledge. How they illuminate the SUMO-dependent Citation: Boulanger, M.; transcriptional mechanisms that have been characterized thus far and how they impact our view of Chakraborty, M.; Tempé, D.; SUMO-dependent chromatin organization are also considered. -
Propionate Hampers Differentiation and Modifies Histone Propionylation and Acetylation in Skeletal Muscle Cells
Mechanisms of Ageing and Development 196 (2021) 111495 Contents lists available at ScienceDirect Mechanisms of Ageing and Development journal homepage: www.elsevier.com/locate/mechagedev Propionate hampers differentiation and modifies histone propionylation and acetylation in skeletal muscle cells Bart Lagerwaard a,b, Marjanne D. van der Hoek a,c,d, Joris Hoeks e, Lotte Grevendonk b,e, Arie G. Nieuwenhuizen a, Jaap Keijer a, Vincent C.J. de Boer a,* a Human and Animal Physiology, Wageningen University and Research, PO Box 338, 6700 AH, Wageningen, the Netherlands b TI Food and Nutrition, P.O. Box 557, 6700 AN, Wageningen, the Netherlands c Applied Research Centre Food and Dairy, Van Hall Larenstein University of Applied Sciences, Leeuwarden, the Netherlands d MCL Academy, Medical Centre Leeuwarden, Leeuwarden, the Netherlands e Department of Nutrition and Movement Sciences, NUTRIM School for Nutrition and Translational Research in Metabolism, Maastricht University, 6200 MD, Maastricht, the Netherlands ARTICLE INFO ABSTRACT Keywords: Protein acylation via metabolic acyl-CoA intermediates provides a link between cellular metabolism and protein Propionylation functionality. A process in which acetyl-CoA and acetylation are fine-tuned is during myogenic differentiation. Skeletal muscle differentiation However, the roles of other protein acylations remain unknown. Protein propionylation could be functionally Histone acylation relevant because propionyl-CoA can be derived from the catabolism of amino acids and fatty acids and was Aging shown to decrease during muscle differentiation. We aimed to explore the potential role of protein propiony lation in muscle differentiation, by mimicking a pathophysiological situation with high extracellular propionate which increases propionyl-CoA and protein propionylation, rendering it a model to study increased protein propionylation. -
Profiling Post-Translational Modifications of Histones in Human
Olszowy et al. Proteome Science (2015) 13:24 DOI 10.1186/s12953-015-0080-7 RESEARCH Open Access Profiling post-translational modifications of histones in human monocyte-derived macrophages Pawel Olszowy1,2, Maire Rose Donnelly1, Chanho Lee1 and Pawel Ciborowski1* Abstract Background: Histones and their post-translational modifications impact cellular function by acting as key regulators in the maintenance and remodeling of chromatin, thus affecting transcription regulation either positively (activation) or negatively (repression). In this study we describe a comprehensive, bottom-up proteomics approach to profiling post-translational modifications (acetylation, mono-, di- and tri-methylation, phosphorylation, biotinylation, ubiquitination, citrullination and ADP-ribosylation) in human macrophages, which are primary cells of the innate immune system. As our knowledge expands, it becomes more evident that macrophages are a heterogeneous population with potentially subtle differences in their responses to various stimuli driven by highly complex epigenetic regulatory mechanisms. Methods: To profile post-translational modifications (PTMs) of histones in macrophages we used two platforms of liquid chromatography and mass spectrometry. One platform was based on Sciex5600 TripleTof and the second one was based on VelosPro Orbitrap Elite ETD mass spectrometers. Results: We provide side-by-side comparison of profiling using two mass spectrometric platforms, ion trap and qTOF, coupled with the application of collisional induced and electron transfer dissociation. We show for the first time methylation of a His residue in macrophages and demonstrate differences in histone PTMs between those currently reported for macrophage cell lines and what we identified in primary cells. We have found a relatively low level of histone PTMs in differentiated but resting human primary monocyte derived macrophages. -
Prediction of Lysine Phosphoglycerylation Based on Residue Adjacency Matrix
G C A T T A C G G C A T genes Article RAM-PGK: Prediction of Lysine Phosphoglycerylation Based on Residue Adjacency Matrix 1, , 1,2,3, , 4,5 Abel Avitesh Chandra * y, Alok Sharma * y , Abdollah Dehzangi and Tatushiko Tsunoda 2,6,7 1 School of Engineering & Physics, University of the South Pacific, Laucala Bay, Suva, Fiji 2 Laboratory for Medical Science Mathematics, RIKEN Center for Integrative Medical Sciences, Yokohama 230-0045, Japan 3 Institute for Integrated and Intelligent Systems, Griffith University, Brisbane, QLD 4111, Australia 4 Department of Computer Science, Rutgers University, Camden, NJ 08102, USA; [email protected] 5 Center for Computational and Integrative Biology, Rutgers University, Camden, NJ 08102, USA 6 Laboratory for Medical Science Mathematics, Department of Biological Sciences, Graduate School of Science, The University of Tokyo, Tokyo 113-0033, Japan; [email protected] 7 Department of Medical Science Mathematics, Medical Research Institute, Tokyo Medical and Dental University, Tokyo 113-8510, Japan * Correspondence: [email protected] (A.A.C.); alok.sharma@griffith.edu.au (A.S.) These authors contributed equally to this work. y Received: 30 September 2020; Accepted: 16 December 2020; Published: 20 December 2020 Abstract: Background: Post-translational modification (PTM) is a biological process that is associated with the modification of proteome, which results in the alteration of normal cell biology and pathogenesis. There have been numerous PTM reports in recent years, out of which, lysine phosphoglycerylation has emerged as one of the recent developments. The traditional methods of identifying phosphoglycerylated residues, which are experimental procedures such as mass spectrometry, have shown to be time-consuming and cost-inefficient, despite the abundance of proteins being sequenced in this post-genomic era. -
The Proteomics Cookbook
Back to website The Proteomics Cookbook A user-friendly guide on what the Proteomics Core can do for you by Simone Sidoli & the Proteomics Core Beta version 0.6 (August 2020) Index Chapter Page number Welcome 3 Standard identification and quantification of a protein mixture 4 Identification and quantification of selected gel bands 6 Identification and quantification of proteins in detergents, e.g. immunoprecipitations 8 Time-resolved proteomics 10 Comprehensive quantification of histone post-translational modifications 12 Quantification of synthesis/degradation rate of proteomes using pulsed metabolic labeling 14 Protein – RNA interaction analysis to identify nucleic acid binding domains of proteins 16 Identification of protein complexes associated on the chromatin (ChIP-SICAP) 18 Estimation of the turnover rate of post-translational modifications 20 Phosphoproteomics 22 Page 2 Introduction & User guide Welcome! The Proteomics Cookbook is a collection of protocols and ideas of experiments that can be performed with the Proteomics Core. Each “recipe” describes established and robust protocols that provide quantitative data about the proteome of your system. This includes identification and quantification of proteins, but also other interesting aspect of your proteome such as turnover, localization and more. You will notice that each protocol is flanked by a page displaying data graphs. The goal is to assist brainstorming regarding the type of information that are achievable with your experiment. We hope this will help you select the most appropriate protocol and stimulate your creativity, including planning novel experiments that you did not expect were feasible. You are more than welcome to design other experiments not described here; we love new challenges! The members of the Core are here at Einstein to help you and discuss with you potential limitations. -
Proteomic Characterization of Novel Histone Post-Translational Modifications Anna M Arnaudo1,2 and Benjamin a Garcia1*
Arnaudo and Garcia Epigenetics & Chromatin 2013, 6:24 http://www.epigeneticsandchromatin.com/content/6/1/24 REVIEW Open Access Proteomic characterization of novel histone post-translational modifications Anna M Arnaudo1,2 and Benjamin A Garcia1* Abstract Histone post-translational modifications (PTMs) have been linked to a variety of biological processes and disease states, thus making their characterization a critical field of study. In the last 5 years, a number of novel sites and types of modifications have been discovered, greatly expanding the histone code. Mass spectrometric methods are essential for finding and validating histone PTMs. Additionally, novel proteomic, genomic and chemical biology tools have been developed to probe PTM function. In this snapshot review, proteomic tools for PTM identification and characterization will be discussed and an overview of PTMs found in the last 5 years will be provided. Keywords: Histone post-translational modifications, Mass spectrometry, Proteomics, Epigenetics Review lysines [6,7]. The recruitment or repulsion of these pro- Introduction teins impacts downstream processes. The idea that PTMs Nearly 50 years ago Vincent Allfrey described histone constitute a code that is read by effector proteins is the acetylation [1]. Since then research has been focused on basis for the histone code hypothesis [8,9]. Mass spec- identifying and mapping a growing list of histone post- trometry (MS) has become an essential tool for deci- translational modifications (PTMs), including lysine phering this code, in part by identifying novel PTMs. In acetylation, arginine and lysine methylation, phosphor- this review we will focus on MS and proteogenomic ylation, proline isomerization, ubiquitination (Ub), ADP methods involved in identifying and characterizing novel ribosylation, arginine citrullination, SUMOylation, car- sites and types of histone PTMs. -
Lysine Acetyltransferase 8 Is Involved in Cerebral Development and Syndromic Intellectual Disability
The Journal of Clinical Investigation RESEARCH ARTICLE Lysine acetyltransferase 8 is involved in cerebral development and syndromic intellectual disability Lin Li,1 Mohammad Ghorbani,1 Monika Weisz-Hubshman,2,3,4 Justine Rousseau,5 Isabelle Thiffault,6,7 Rhonda E. Schnur,8,9 Catherine Breen,10 Renske Oegema,11 Marjan M.M. Weiss,12 Quinten Waisfisz,12 Sara Welner,13 Helen Kingston,10 Jordan A. Hills,14 Elles M.J. Boon,12 Lina Basel-Salmon,2,3,4,15 Osnat Konen,4,16 Hadassa Goldberg-Stern,4,17 Lily Bazak,3,4 Shay Tzur,18,19 Jianliang Jin,1,20 Xiuli Bi,1 Michael Bruccoleri,1 Kirsty McWalter,9 Megan T. Cho,9 Maria Scarano,8 G. Bradley Schaefer,14 Susan S. Brooks,13 Susan Starling Hughes,6,7 K.L.I. van Gassen,11 Johanna M. van Hagen,12 Tej K. Pandita,21 Pankaj B. Agrawal,22 Philippe M. Campeau,5 and Xiang-Jiao Yang1,23 1Rosalind and Morris Goodman Cancer Research Centre and Department of Medicine, McGill University, Montreal, Quebec, Canada. 2Pediatric Genetics Unit, Schneider Children’s Medical Center of Israel, Petach Tikva, Israel. 3Raphael Recanati Genetic Institute, Rabin Medical Center, Petach Tikva, Israel. 4Sackler Faculty of Medicine, Tel Aviv University, Tel Aviv, Israel. 5Paediatric Department, CHU Sainte- Justine Hospital, University of Montreal, Quebec, Canada. 6Center for Pediatric Genomic Medicine & Division of Clinical Genetics, Children’s Mercy Hospital, Kansas City, Missouri, USA. 7Faculty of Medicine, University of Missouri-Kansas City, Kansas City, Missouri, USA. 8Division of Genetics, Cooper University Health Care, Camden, New Jersey, USA. 9GeneDx, Gaithersburg, Maryland, USA. -
Dietary Propionate Induces Intestinal Oxidative Stress Via Inhibition Of
bioRxiv preprint doi: https://doi.org/10.1101/2020.08.10.245399; this version posted August 11, 2020. The copyright holder for this preprint (which was not certified by peer review) is the author/funder, who has granted bioRxiv a license to display the preprint in perpetuity. It is made available under aCC-BY-NC-ND 4.0 International license. 1 Dietary propionate induces intestinal oxidative stress via inhibition of 2 SIRT3-mediated SOD2 depropionylation 3 Qian-wen Ding1,2, Zhen Zhang3, Yu Li1, Hong-liang Liu1, Qiang Hao1, Ya-lin Yang3, Einar Ringø2, 4 Rolf Erik Olsen2, Jihong Liu Clarke 4, Chao Ran3, #, Zhi-gang Zhou1,# 5 1 China-Norway Joint Lab on Fish Gastrointestinal Microbiota, Feed Research Institute, Chinese 6 Academy of Agricultural Sciences, Beijing 100081, China 7 2 Norway-China Joint Lab on Fish Gastrointestinal Microbiota, Institute of Biology, Norwegian 8 University of Science and Technology, Trondheim, Norway 9 3 Key Laboratory for Feed Biotechnology of the Ministry of Agriculture, Feed Research Institute, 10 Chinese Academy of Agricultural Sciences, Beijing 100081, China 11 4 NIBIO, Norwegian Institute of Bioeconomy Research, 1431 Ås, Norway 12 # Correspondence and requests for materials should be addressed to Chao Ran (email: 13 [email protected]) and Zhi-gang Zhou (email: [email protected]). bioRxiv preprint doi: https://doi.org/10.1101/2020.08.10.245399; this version posted August 11, 2020. The copyright holder for this preprint (which was not certified by peer review) is the author/funder, who has granted bioRxiv a license to display the preprint in perpetuity. It is made available under aCC-BY-NC-ND 4.0 International license. -
SET Domain–Mediated Lysine Methylation Controls Growth in Lower Organisms and Transcriptional Machinery in Hosts
CORE Metadata, citation and similar papers at core.ac.uk Provided by UCL Discovery SET domain–mediated lysine methylation controls growth in lower organisms and transcriptional machinery in hosts Chukwuazam Nwasike1, Sinead Ewert2, Srdan Jovanovic2, Shozeb Haider,2,a and Shiraz Mujtaba1,a 1City University of New York, Medgar Evers College, Brooklyn, NY. 2UCL School of Pharmacy, University College London, London, UK Correspondence should be addressed to: Shozeb Haider, UCL School of Pharmacy, University College London, London, WC1N 1AX, UK. [email protected]. Shiraz Mujtaba, City University of New York, Medgar Evers College, Brooklyn, NY 11225. [email protected] Running title: SET protein–mediated methylation Keywords: epigenetics; SET protein; methyl-transferase; chromatin; histone proteins; lysine methylation Abstract Su(var)3-9, Enhancer-of-zeste, Trithorax (SET) domain–mediated lysine methylation, one of the major epigenetic marks, has been found to regulate chromatin-mediated gene transcription. Published studies have established, further, that methylation is not restricted to nuclear proteins but is involved in many cellular processes, including growth, differentiation, immune regulation, and cancer progression. The biological complexity of lysine methylation emerges from its capacity to cause gene activation or gene repression owing to the specific position of methylated- lysine moieties on the chromatin. Accumulating evidence suggests that despite the absence of chromatin, viruses and prokaryotes also express SET domain–containing proteins, although their functional roles remain under investigated. One possibility is that SET domain–containing proteins in lower organisms have more than one biological function, for example in regulating lower organism growth or in manipulating host transcription machinery in order to establish infection. -
PAF Fall 2018 Newsletter
SEARCHING FOR A CURE HOPE FOR OUR CHILDREN Propionic Acidemia Foundation VOLUME 1, ISSUE 25 FALL 2018 PA Registry PAF Warrior Wisdom Conference Help move research forward for propionic October 19-21, 2018 acidemia. Participate in Nationwide Hotel & Conference Center the Propionic Acidemia 100 Green Meadows Drive South, Lewis Center, OH 43035 International Regis- try. As of September 1st, there are 64 partic- Friday, October 19th ipants. For more infor- 6:30pm-8:30pm Registration, Reception with mation on joining the light snacks, New Parent Orientation registry, or to update Saturday, October 20th your information, go to 8:00am-8:30am Registration www.paregistry.org. 8:30am-5:00pm Conference– Presentations, Family Panel, and Visit Exhibitors. Topics Include: Propionic Acidemia, Secondary Complications, Nutrition Management and INSIDE Guidelines, Nutrition during Menstruation and Pregnancy, PAF Updates (detailed agenda to be posted on website) NIH GRANT & NOVEL 2 5:00pm-9:00pm Dinner, Social & Networking Time THERAPIES FOR PA Sunday, October 21st JOHNS HOPKINS 3 Run for PAF or cheer on participants in the Nationwide Children’s Hospital Columbus UNIVERSITY RESEARCH Marathon/Half Marathon! (Separate Registration) Location and directions for cheer- ing on our PAF Runners available at the conference. LIVER TRANSPLANT OUTCOMES: 4-5 HOTEL INFORMATION PART 2 Special Group Rate: $119.00 plus taxes, includes breakfast. PAF EVENT & FUND- 6 Please call or email Kelsey McClincy at (614) 602-0114 or RAISING SPOTLIGHT [email protected] Monday through Friday from 7:30 am- 4:00 pm to make your reservation. Reserve your room GRACE-MARIE’S STORY 6 before they sell out or by September 20th whichever comes first.