Thermodynamic Stability of Hydrogen Clathrates
Total Page:16
File Type:pdf, Size:1020Kb
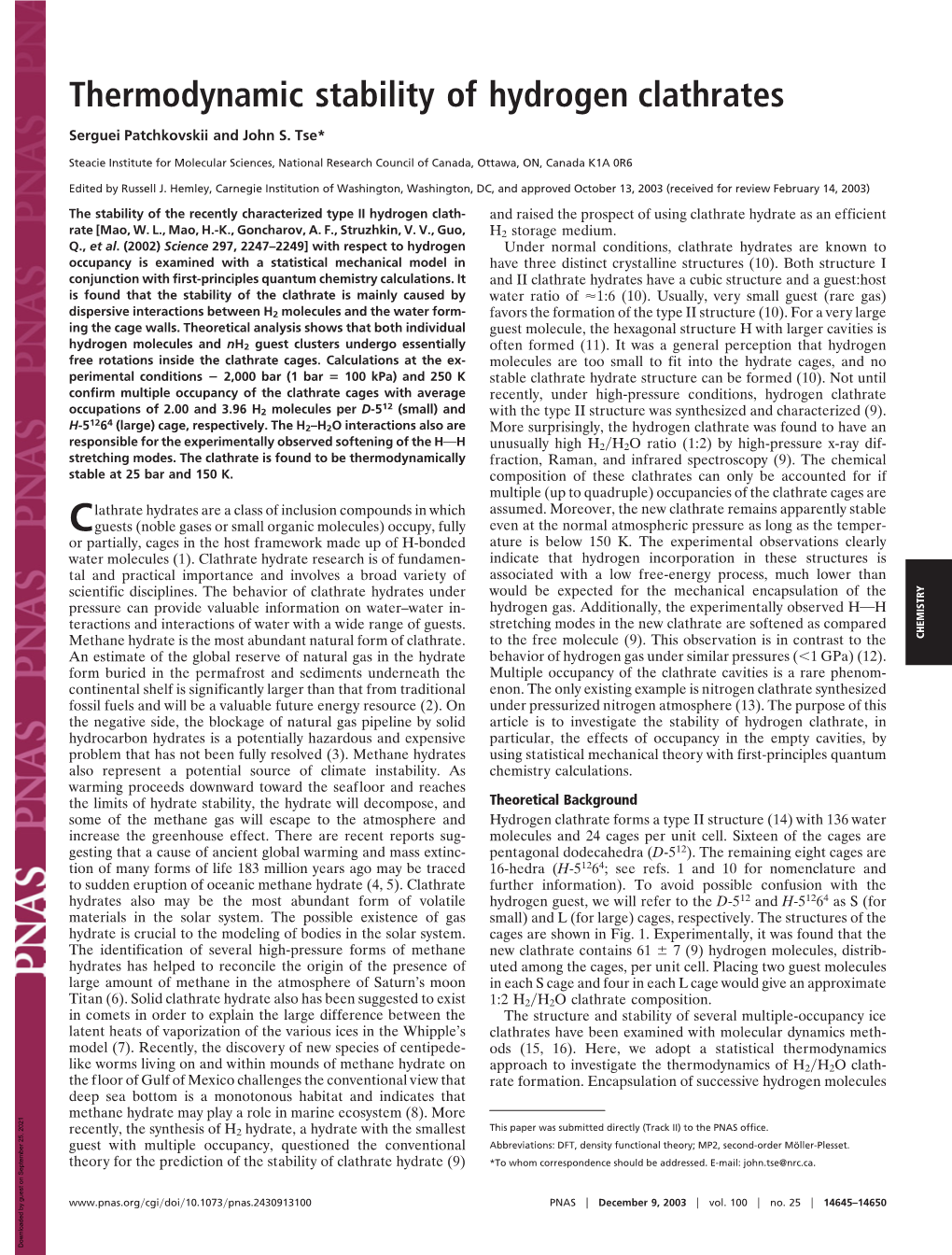
Load more
Recommended publications
-
Effects of Pressurized Argon and Krypton Treatments on the Quality of Fresh White Mushroom (Agaricus Bisporus)
Food and Nutrition Sciences, 2013, 4, 1191-1200 Published Online December 2013 (http://www.scirp.org/journal/fns) http://dx.doi.org/10.4236/fns.2013.412153 Effects of Pressurized Argon and Krypton Treatments on the Quality of Fresh White Mushroom (Agaricus bisporus) Camel Lagnika1,2, Min Zhang1*, Mohanad Bashari1, Fatoumata Tounkara1 1State Key Laboratory of Food Science and Technology, School of Food Science and Technology, Jiangnan University, Wuxi, China; 2Ecole Nationale des Sciences et Technique de Conservation et Transformation des Produits Agricoles de Sakété, Université d’Agri- culture de Kétou, Kétou, Benin. Email: *[email protected], [email protected] Received August 26th, 2013; revised September 26th, 2013; accepted October 4th, 2013 Copyright © 2013 Camel Lagnika et al. This is an open access article distributed under the Creative Commons Attribution License, which permits unrestricted use, distribution, and reproduction in any medium, provided the original work is properly cited. In accor- dance of the Creative Commons Attribution License all Copyrights © 2013 are reserved for SCIRP and the owner of the intellectual property Camel Lagnika et al. All Copyright © 2013 are guarded by law and by SCIRP as a guardian. ABSTRACT Effects of argon, krypton and their mixed pressure treatments on the quality of white mushrooms were studied during 9 days of storage at 4˚C. Among all treatments in this study, the minimum respiration rate, polyphenoloxidase activity, retained color change, antioxidants and delayed pseudomonas growth were observed with pressure argon (5 MPa) fol- lowed by mixing argon and krypton (2.5 MPa each) treatments. Respiration rates after 9 days of storage were 5.35%, 6.20%, 7.50%, 7.60%, 7.91% and 8.95% for HA5, HAK, HA2, HK5, HK2 and control, respectively. -
Thermodynamics of Clathrate Hydrate at Low and High Pressures With
THE ASTROPHYSICAL JOURNAL SUPPLEMENT SERIES, 58:493-531, 1985 July © 1985. The American Astronomical Society. All rights reserved. Printed in U.S.A. THERMODYNAMICS OF CLATHRATE HYDRATE AT LOW AND HIGH PRESSURES 1985ApJS...58..493L WITH APPLICATION TO THE OUTER SOLAR SYSTEM1 JONATHAN I. LUNINE2 AND DAVID J. STEVENSON Division of Geological and Planetary Sciences, California Institute of Technology Received 1984 June 29; accepted 1985 January 10 ABSTRACT The thermodynamic stability of clathrate hydrate is calculated under a wide range of temperature and pressure conditions applicable to solar system problems, using a statistical mechanical theory developed by van der Waals and Platteeuw (1959) and existing experimental data on properties of clathrate hydrates and their components. At low pressure, dissociation pressures and partition functions (Langmuir constants) for CO clathrate (hydrate) have been predicted, using the properties of clathrate containing, as guests, molecules similar to CO. The comparable or higher propensity of CO to incorporate in clathrate relative to N2 is used to argue for high CO-to-N2 ratios in primordial Titan if N2 was accreted as clathrate. The relative incorporation of noble gases in clathrate ~rom a solar composition gas at low temperatures is calculated and applied to the case of giant-planet atmospheres and icy satellites. It is argued that nonsolar but well-constrained noble gas abundances will be measured by Galileo in the Jovian atmosphere if the observed carbon enhancement is due to bombardment of the atmosphere by clathrate-bearing planetesimals sometime after planetary formation. The noble gas abun dances in Titan's atmosphere are also predicted under the hypothesis that much of the satellite's methane accreted as clathrate. -
Effects of Highpressure Argon and Nitrogen Treatments on Respiration
Research Article Received: 6 March 2011 Revised: 20 October 2011 Accepted: 21 December 2011 Published online in Wiley Online Library: 24 February 2012 (wileyonlinelibrary.com) DOI 10.1002/jsfa.5612 Effects of high-pressure argon and nitrogen treatments on respiration, browning and antioxidant potential of minimally processed pineapples during shelf life Zhi-shuang Wu,a Min Zhanga∗ and Shao-jin Wangb Abstract BACKGROUND: High-pressure (HP) inert gas processing causes inert gas and water molecules to form clathrate hydrates that restrict intracellular water activity and enzymatic reactions. This technique can be used to preserve fruits and vegetables. In this study, minimally processed (MP) pineapples were treated with HP (∼10 MPa) argon (Ar) and nitrogen (N) for 20 min. The effects of these treatments on respiration, browning and antioxidant potential of MP pineapples were investigated after cutting and ◦ during 20 days of storage at 4 C. RESULTS: Lower respiration rate and ethylene production were found in HP Ar- and HP N-treated samples compared with control samples. HP Ar and HP N treatments effectively reduced browning and loss of total phenols and ascorbic acid and maintained antioxidant capacity of MP pineapples. They did not cause a significant decline in tissue firmness or increase in juice leakage. HP Ar treatments had greater effects than HP N treatments on reduction of respiration rate and ethylene production and maintenance of phenolic compounds and DPPH• and ABTS•+ radical-scavenging activities. ◦ CONCLUSION: Both HP Ar and -
Low Temperature Calorimetric Studies a Thesis Submitted for the Degree of Doctor of Philosophy of the University of London
"Low Temperature Calorimetric Studies of Inclusion Compounds". A thesis submitted for the Degree of Doctor of Philosophy of the University of London. by ROBERT CHARLES PEMBERTON B.Sc., A.R.C.S. Physical Chemistry (Cryogenics Laboratory), Royal College of Science, Imperial College, London, S.W. 7. March, 1965. 1 ACKNOWTRDGEIVENTS. I wish to express my sincere thanks to Dr. N.G.Parsonage for supervising this work and particularly for his assistance with heat capacity measurements. I am also indebted to the College Technical Staff for their co—operation in the construction of certain items of apparatus and to my fellow students for useful discussions. British Petroleum Ltd., also provided hydrocarbon samples of high purity for which I thank them, and a maintainance grant from the Department of Scientific and Industrial Research over the period 19(11 — 1964 is gratefully acknowledged. Robert C. Pemberton.—) 2 ABSTRACT The heat capacities (Cp) of the n-decane, n-dodecane, n-hexadecane, n-eicosane, n-pentadecane and 2 -methyl-pentadecane adducts of urea have been measured between 12° - 300° K. All these adducts show a small exess heat absorption with a maximum at 16.2 + o.1 K and, with the exception of the 2-methyl-pentadecane compound, a sharp co-operative transition having a maximum in the temperature range 110° to 190°K. The transition temperatures (Tc), enthalpies(AH) and entro- pies (A S) of transitionpar mole of adducted hydrocarbon are reported. These increase with the length of the hydro- carbon chain along smooth curves except for the odd membered C15 H32 homologuefor which Tc is higher and /Ai and CAS lower than expected from the even hydrocarbon series. -
High-Pressure Photodissociation of Water As a Tool for Hydrogen Synthesis and Fundamental Chemistry
High-pressure photodissociation of water as a tool for hydrogen synthesis and fundamental chemistry Matteo Ceppatelli, Roberto Bini1, and Vincenzo Schettino European Laboratory for Non-Linear Spectroscopy, Via N. Carrara 1, I-50019 Sesto Fiorentino, Florence, Italy; and Dipartimento di Chimica, Universita´di Firenze, Via della Lastruccia 3, I-50019 Sesto Fiorentino, Florence, Italy Edited by Russell J. Hemley, Carnegie Institution of Washington, Washington, DC, and approved May 14, 2009 (received for review February 18, 2009) High-pressure methods have been demonstrated to be efficient in hydrocarbons triggered by 2-photon (TP) excitations realized providing new routes for the synthesis of materials of technolog- with cw (continuous wave) low-power laser sources that, because ical interest. In several molecular compounds, the drastic pressure of the small cross-section of TP transitions, ensure catalytic conditions required for spontaneous transformations have been amounts of excited species (5, 6, 12, 15). lowered to the kilobar range by photoactivation of the reactions. Among simple molecular systems, water is of primary impor- At these pressures, the syntheses are accessible to large-volume tance because of its abundance on the Earth‘s surface and applications and are of interest to bioscience, space, and environ- because it is the most abundant polyatomic molecule in the mental chemistry. Here, we show that the short-lived hydroxyl visible universe (16). In addition, chemical reactions taking place radicals, produced in the photodissociation of water molecules by in liquid water are essential for many processes in environmental near-UV radiation at room temperature and pressures of a few science and biology. -
Swri IR&D Program 2013
Internal Research and Development 2013 The SwRI IR&D Program exists to broaden the Institute's technology base and to encourage staff professional growth. Internal funding of research enables the Institute to advance knowledge, increase its technical capabilities, and expand its reputation as a leader in science and technology. The program also allows Institute engineers and scientists to continually grow in their technical fields by providing freedom to explore innovative and unproven concepts without contractual restrictions and expectations. Space Science Materials Research & Structural Mechanics Intelligent Systems, Advanced Computer & Electronic Technology, & Automation Measurement & Nondestructive Evaluation of Materials & Structures Engines, Fuels, Lubricants, & Vehicle Systems Geology & Nuclear Waste Management Fluid & Machinery Dynamics Electronic Systems & Instrumentation Chemistry & Chemical Engineering Copyright© 2014 by Southwest Research Institute. All rights reserved under U.S. Copyright Law and International Conventions. No part of this publication may be reproduced in any form or by any means, electronic or mechanical, including photocopying, without permission in writing from the publisher. All inquiries should be addressed to Communications Department, Southwest Research Institute, P.O. Drawer 28510, San Antonio, Texas 78228-0510, [email protected], fax (210) 522 -3547. 2013 IR&D | IR&D Home SwRI IR&D 2013 – Space Science Capability Development and Demonstration for Next-Generation Suborbital Research, 15-R8115 Development -
Ageing and Langmuir Behavior of the Cage Occupancy in the Nitrogen Gas Hydrate
crystals Article Ageing and Langmuir Behavior of the Cage Occupancy in the Nitrogen Gas Hydrate Claire Petuya 1, Françoise Damay 2, Sarah Desplanche 1, Christian Aupetit 1 and Arnaud Desmedt 1,* ID 1 Institut des Sciences Moléculaire-UMR CNRS 5255-Univ. Bordeaux, 351 cours de la Libération, 33404 Talence CEDEX, France; [email protected] (C.P.); [email protected] (S.D.); [email protected] (C.A.) 2 Laboratoire Léon Brillouin, UMR 12 CEA-CNRS, Bât. 563 CEA Saclays, 91191 Gif-sur-Yvette CEDEX, France; [email protected] * Correspondence: [email protected]; Tel.: +33-540-002-937 Received: 22 February 2018; Accepted: 16 March 2018; Published: 23 March 2018 Abstract: Clathrate hydrates are ice-like systems in which nanometric water cages encapsulate guest molecules. Functionalizing clathrate hydrates is an important issue, accomplished by playing with their chemical composition and their cage structure. In this issue, the cage occupancy and its kinetics constitute key information for future developments. In many aspects, nitrogen gas hydrate represents an interesting system not only for its applied relevance (e.g., gas separation and methane/carbon dioxide exchange), but also for its fundamental interest (e.g., structural metastability and kinetics). Thanks to the complementarity of neutron diffraction and Raman scattering, the vibrational signatures of the so-called SI and SII clathrate structures of the nitrogen hydrates are reviewed. Moreover, the investigation of the ageing of the SII structure is reported together with its interpretation in the frame of the Langmuir behavior of the cage filling at low temperature. -
Cage Occupancies in Nitrogen Clathrate Hydrates from Monte Carlo Simulations
Cage occupancies in nitrogen clathrate hydrates from Monte Carlo simulations Vincent Ballenegger Institut UTINAM, Univ. Bourgogne-Franche-ComtÃľ, UMR CNRS 6213, 16, route de Gray, 25030 BesanÃğon Cedex, France E-mail: [email protected] Abstract Their exploitation could be combined with the sequestration of carbon dioxide, in the form of Comparisons of Gibbs ensemble Monte Carlo CO2 hydrate on the seafloor, to help keeping the simulations with experimental data for the cage concentration of this global warming gas under occupancies in N2 clathrate hydrates are per- control.2 Nitrogen molecules were found to act formed to assess the accuracy of such simu- as a promoter in the N2âĂŞassisted CH4 CO2 lations, to refine the effective potentials em- exchange reaction.3 Since flue gas mixtures con- ployed, and to help interpret recently measured tain a mixture of N2 and CO2, a deep under- large cage over small cage occupancy ratios standing of mixed N2 CO2 clathrates, and also [Petuya et al., J. Phys. Chem. C 122, 566 pure N2 and CO2 clathrates as reference sys- (2018)]. Different sets of interaction potentials tems, is important for this technology. Air for N2 N2,N2 H2O and H2O H2O interac- clathrates, containing N2,O2 and other trace tions are considered. Some of them fail to re- gases, are found on Earth below ice caps at a produce the known experimental fact that some depth of 1000 m or more. They are of particu- large cages are doubly occupied at 273 K and lar interest as they contains relics of our ancient high pressures. -
Uva-DARE (Digital Academic Repository)
UvA-DARE (Digital Academic Repository) Recent advances in the study of high pressure binary systems Schouten, J.A. DOI 10.1088/0953-8984/7/3/004 Publication date 1995 Published in Journal of Physics-Condensed Matter Link to publication Citation for published version (APA): Schouten, J. A. (1995). Recent advances in the study of high pressure binary systems. Journal of Physics-Condensed Matter, 7, 469-482. https://doi.org/10.1088/0953-8984/7/3/004 General rights It is not permitted to download or to forward/distribute the text or part of it without the consent of the author(s) and/or copyright holder(s), other than for strictly personal, individual use, unless the work is under an open content license (like Creative Commons). Disclaimer/Complaints regulations If you believe that digital publication of certain material infringes any of your rights or (privacy) interests, please let the Library know, stating your reasons. In case of a legitimate complaint, the Library will make the material inaccessible and/or remove it from the website. Please Ask the Library: https://uba.uva.nl/en/contact, or a letter to: Library of the University of Amsterdam, Secretariat, Singel 425, 1012 WP Amsterdam, The Netherlands. You will be contacted as soon as possible. UvA-DARE is a service provided by the library of the University of Amsterdam (https://dare.uva.nl) Download date:02 Oct 2021 I. Phys.: Condens. Matter 7 (1995) 469482. Printed in the UK REVIEW ARTICLE Recent advances in the study of high-pressure binary systems J A Schouten Van der Waals-Zeemanlabntory, University of Amsterdam, Valckeniersfnar 65, 1018 XE Amsterdam The Netherlands .Received 31 October 1994. -
The Astrophysical Journal Supplement Series, 58:493-531
The Astrophysical Journal Supplement Series, 58:493-531,1985 July .493L © 1985. The American Astronomical Society. All rights reserved. Printed in U.S.A. 58. ... THERMODYNAMICS OF CLATHRATE HYDRATE AT LOW AND HIGH PRESSURES 1985ApJS WITH APPLICATION TO THE OUTER SOLAR SYSTEM1 Jonathan I. Lunine2 and David J. Stevenson Division of Geological and Planetary Sciences, California Institute of Technology Received 1984 June 29; accepted 1985 January 10 ABSTRACT The thermodynamic stability of clathrate hydrate is calculated under a wide range of temperature and pressure conditions applicable to solar system problems, using a statistical mechanical theory developed by van der Waals and Platteeuw (1959) and existing experimental data on properties of clathrate hydrates and their components. At low pressure, dissociation pressures and partition functions (Langmuir constants) for CO clathrate (hydrate) have been predicted, using the properties of clathrate containing, as guests, molecules similar to CO. The comparable or higher propensity of CO to incorporate in clathrate relative to N2 is used to argue for high CO-to-N2 ratios in primordial Titan if N2 was accreted as clathrate. The relative incorporation of noble gases in clathrate from a solar composition gas at low temperatures is calculated and applied to the case of giant-planet atmospheres and icy satellites. It is argued that nonsolar but well-constrained noble gas abundances will be measured by Galileo in the Jovian atmosphere if the observed carbon enhancement is due to bombardment of the atmosphere by clathrate-bearing planetesimals sometime after planetary formation. The noble gas abun- dances in Titan’s atmosphere are also predicted under the hypothesis that much of the satellite’s methane accreted as clathrate. -
Crystallization of Mixed Clathrate Hydrates Under Equilibrium and Non-Equilibrium Conditions : Pvtx Measurements and Thermodynamic Modeling Saheb Maghsoodloobabakhani
Crystallization of mixed clathrate hydrates under equilibrium and non-equilibrium conditions : PVTx measurements and thermodynamic modeling Saheb Maghsoodloobabakhani To cite this version: Saheb Maghsoodloobabakhani. Crystallization of mixed clathrate hydrates under equilibrium and non-equilibrium conditions : PVTx measurements and thermodynamic modeling. Other. Université de Lyon, 2018. English. NNT : 2018LYSEM027. tel-02885353 HAL Id: tel-02885353 https://tel.archives-ouvertes.fr/tel-02885353 Submitted on 30 Jun 2020 HAL is a multi-disciplinary open access L’archive ouverte pluridisciplinaire HAL, est archive for the deposit and dissemination of sci- destinée au dépôt et à la diffusion de documents entific research documents, whether they are pub- scientifiques de niveau recherche, publiés ou non, lished or not. The documents may come from émanant des établissements d’enseignement et de teaching and research institutions in France or recherche français ou étrangers, des laboratoires abroad, or from public or private research centers. publics ou privés. N°d’ordre NNT : 2018LYSEM027 THESE de DOCTORAT DE L’UNIVERSITE DE LYON opérée au sein de l’Ecole des Mines de Saint-Etienne Ecole Doctorale N° 488 Sciences, Ingénierie, Santé Spécialité de doctorat : Génie des Procédés Discipline : DS8 Sciences pour l'ingénieur Soutenue prévue le 14/12/2018, par : Saheb Maghsoodloo Babakhani Cristallisation à l'équilibre et hors équilibre d'hydrates mixtes de gaz : Mesures PVTx et modélisation thermodynamique Crystallization of mixed clathrate hydrates under equilibrium and non-equilibrium conditions: PVTx measurements and thermodynamic modeling Devant le jury composé de : Christelle Miqueu Maître de Conférences HDR, Université De Pau Et Des Pays De Présidente L'adour, France Karine Ballerat-Busserolles Ingénieure de Recherche - CNRS, Institut de Chimie de Clermont- Rapporteure Ferrand, France. -
1 Exoplanet Chemistry
1 Exoplanet Chemistry Katharina Lodders Planetary Chemistry Laboratory, Dept. Of Earth & Planetary Sciences and McDonnell Center for the Space Sciences, Washington University, One Brookings Drive, Saint Louis, MO 63130 Abstract: The characteristic chemistry of terrestrial planets and, in particular, of giant planets rich and poor in He and H2 are described. Keywords: terrestrial planets, gas giant planets, planet formation, planet compositions 1.1 A Goodly Gallery of Planets The current state of observations on the chemical nature of exoplanets is comparable to that of the early days of solar system planetary science, when not much more than the orbits and densities of planets were known. Information about exoplanet chemical compositions from direct observations is still limited. However, chemical and physical observations and models for planets in our solar system developed since the 1950s should provide the tools to understand other planets, if models for solar system planets are indeed correct. As such, the observational data to come in the following years undoubtedly will test the fine-tuned planetary formation and evolution models that seem to work well for understanding the planets in our solar system. There are four broad basic types in our gallery of planets in the solar system and very likely in most of the other planetary systems. The reason for this is the first order similarity of the relative elemental abundances of most stars in the galactic disk and the compounds that the elements can form. Planets originate from the materials in a stellar accretion disk that had a similar composition as the final star itself. This limits the possible chemical nature of the building blocks of planets, despite the wide range of possible physical properties in the different accretion disks around other stars.