1 Efficient Green Methanol Synthesis from Glycerol Muhammad H. Haider
Total Page:16
File Type:pdf, Size:1020Kb
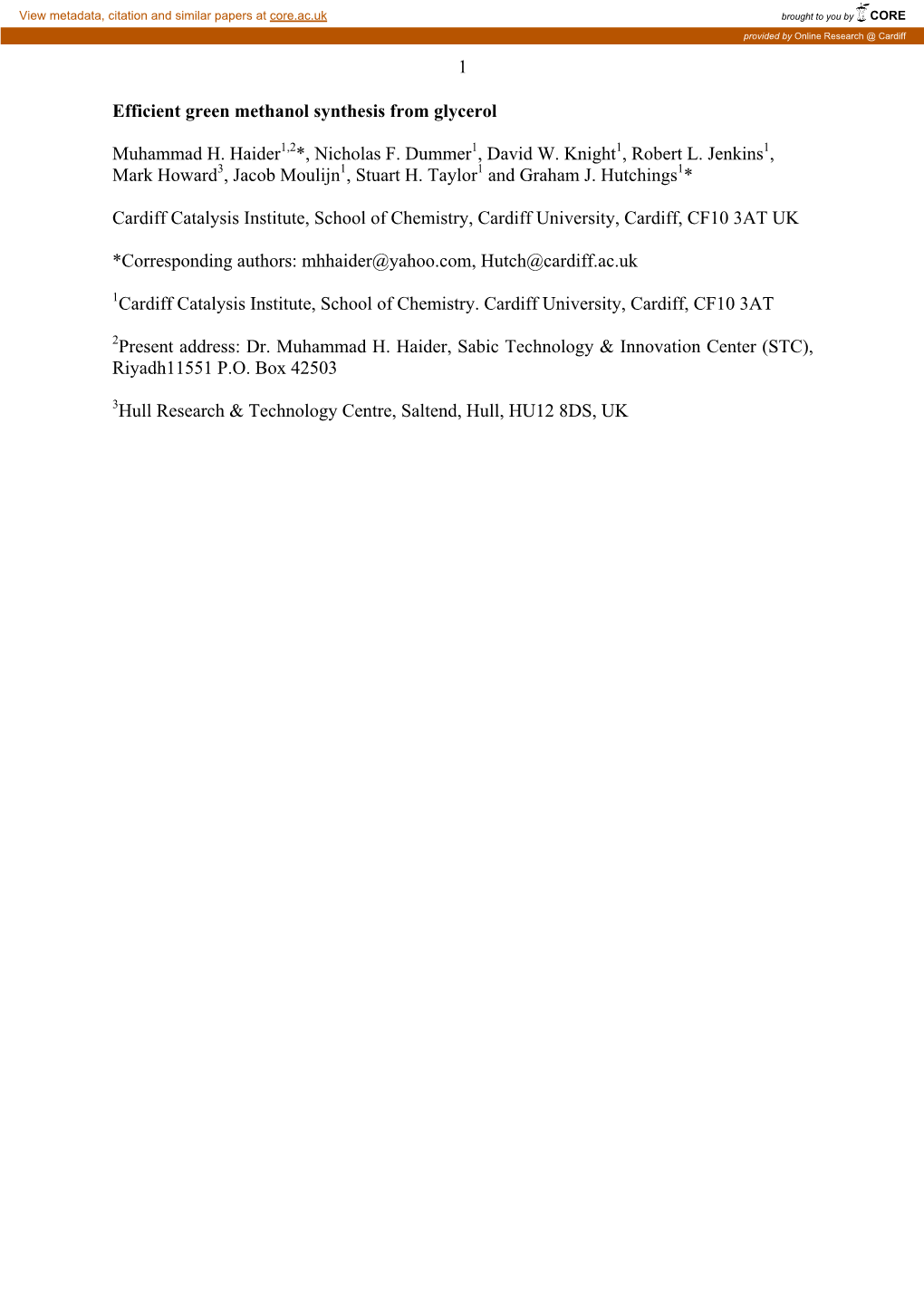
Load more
Recommended publications
-
Bio-Butanol Production from Glycerol with Clostridium
Lin et al. Biotechnol Biofuels (2015) 8:168 DOI 10.1186/s13068-015-0352-6 RESEARCH Open Access Bio‑butanol production from glycerol with Clostridium pasteurianum CH4: the effects of butyrate addition and in situ butanol removal via membrane distillation De‑Shun Lin1, Hong‑Wei Yen2, Wei‑Chen Kao1, Chieh‑Lun Cheng1, Wen‑Ming Chen3, Chieh‑Chen Huang4 and Jo‑Shu Chang1,4,5* Abstract Background: Clostridium pasteurianum CH4 was used to produce butanol from glycerol. The performance of butanol fermentation was improved by adding butyrate as the precursor to trigger the metabolic pathway toward butanol production, and by combining this with in situ butanol removal via vacuum membrane distillation (VMD) to avoid the product inhibition arising from a high butanol concentration. 1 Results: Adding 6 g L− butyrate as precursor led to an increase in the butanol yield from 0.24 to 0.34 mol butanol 1 (mol glycerol)− . Combining VMD and butyrate addition strategies could further enhance the maximum effective 1 1 butanol concentration to 29.8 g L− , while the yield was also improved to 0.39 mol butanol (mol glycerol)− . The butanol concentration in the permeate of VMD was nearly five times higher than that in the feeding solution. Conclusions: The proposed butyrate addition and VMD in situ butanol removal strategies are very effective in enhancing both butanol titer and butanol yield. This would significantly enhance the economic feasibility of fermen‑ tative production of butanol. The VMD-based technology not only alleviates the inhibitory effect of butanol, but also markedly increases butanol concentration in the permeate after condensation, thereby making downstream process‑ ing easier and more cost-effective. -
A Cooked Composition of Glycerine and Hydrogenated Starch Hydrolysate and the Use Thereof As a Stabilizer for L-Aspartic Acid Sweetening Agent in Comestibles
Europaisches Patentamt 0 323 442 J> European Patent Office Publication number: A1 Office europeen des brevets EUROPEAN PATENT APPLICATION @ Application number: 89102633.8 IntCI* A 23 L 1/236 A 23 G 3/30 @ Date of filing: 27.03.86 2g) Priority: 29.03.85 US 717630 © Applicant: NABISCO BRANDS, INC. 100 DeForest Avenue East Hanover New Jersey 07936 (US) §) Date of publication of application: 05.07.89 Bulletin 89/27 @ Inventor: Carroll, Thomas J. States: BE DE FR GB IT 20-30 43rd Street g) Designated Contracting Astoria, N.Y. (US) jig) Publication number of the earlier application in Kehoe, Gary S. accordance with Art. 76 EPC: 0 196 640 10 Cross Hill Road Ridgefield, Conn. (US) @) Representative : Brauns, Hans-Adolf, Dr. rer. nat. et al Hoffmann, Eitle & Partner, Patentanwalte Arabellastrasse 4 D-8000 Munich 81 (DE) @ A cooked composition of glycerine and hydrogenated starch hydrolysate and the use thereof as a stabilizer for L-aspartic acid sweetening agent in comestibles. (g) A cooked composition of glycerine and hydrogenated starch hydrolysate having a moisture content of 10 ± 6% (W/W). Said composition can be used as a stabilizer for L-aspartic acid sweetening agent in comestibles. CM CO CM eo a. LU Bundesdruckerei Berlin EP 0 323 442 A1 Description A COOKED COMPOSITION OF GLYCERINE AND HYDROGENATED STARCH HYDROLYSATE AND THE USE THEREOF AS A STABILIZER FOR L-ASPARTIC ACID SWEETENING AGENT IN COMESTIBLES Aspartame which is used extensively in many types of sugarless foodstuffs, or other comestible products, 5 such as chewing gum, is known to readily decompose in the presence of moisture into decomposition products such as diketopiperizine which causes a significant loss in the sweetness properties of such products during their shelf lives. -
Intensified Crude Glycerol Conversion to Butanol by Immobilized Clostridium Pasteurianum
Intensified crude glycerol conversion to butanol by immobilized Clostridium pasteurianum Krasňan, V., Plž, M., Marr, A. C., Markošová, K., Rosenberg, M., & Rebros, M. (2018). Intensified crude glycerol conversion to butanol by immobilized Clostridium pasteurianum. Biochemical Engineering Journal. https://doi.org/10.1016/j.bej.2018.03.005 Published in: Biochemical Engineering Journal Document Version: Peer reviewed version Queen's University Belfast - Research Portal: Link to publication record in Queen's University Belfast Research Portal General rights Copyright for the publications made accessible via the Queen's University Belfast Research Portal is retained by the author(s) and / or other copyright owners and it is a condition of accessing these publications that users recognise and abide by the legal requirements associated with these rights. Take down policy The Research Portal is Queen's institutional repository that provides access to Queen's research output. Every effort has been made to ensure that content in the Research Portal does not infringe any person's rights, or applicable UK laws. If you discover content in the Research Portal that you believe breaches copyright or violates any law, please contact [email protected]. Download date:28. Sep. 2021 1 Intensified crude glycerol conversion to butanol by immobilized Clostridium 2 pasteurianum 3 4 Vladimír Krasňan1, Michal Plž1, Andrew C. Marr2, Kristína Markošová1, Michal 5 Rosenberg1, Martin Rebroš1* 6 1Institute of Biotechnology, Slovak University of Technology, Radlinského 9, 812 37 7 Bratislava, Slovakia 8 2School of Chemistry and Chemical Engineering, Queen’s University of Belfast, UK. 9 *corresponding author ([email protected]; Tel: +421 2 59 325 480) 10 1 11 Abstract 12 Butanol production from glycerol was investigated through Clostridium 13 pasteurianum entrapped into polyvinyl alcohol particles. -
Biovalorisation of Crude Glycerol and Xylose Into Xylitol by Oleaginous Yeast Yarrowia Lipolytica
Biovalorisation of crude glycerol and xylose into xylitol by oleaginous yeast Yarrowia lipolytica Ashish Prabhu Craneld University Dominic J Thomas Craneld University Rodrigo Ledesma- Amaro Imperial College London Gary A Leeke University of Birmingham Angel Medina Vaya Craneld University Carol Verheecke-Vaessen Craneld University Frederic Coulon Craneld University Vinod Kumar ( [email protected] ) Craneld University https://orcid.org/0000-0001-8967-6119 Research Keywords: Glycerol, Xylose, Yarrowia lipolytica, Biotransformation, Xylitol Posted Date: March 26th, 2020 DOI: https://doi.org/10.21203/rs.3.rs-19009/v1 License: This work is licensed under a Creative Commons Attribution 4.0 International License. Read Full License Version of Record: A version of this preprint was published at Microbial Cell Factories on June 3rd, 2020. See the published version at https://doi.org/10.1186/s12934-020-01378-1. Page 1/28 Abstract Background: Xylitol is a commercially important chemical with multiple applications in food and pharmaceutical industries. According to the US Department of Energy, xylitol is among the twelve platform chemicals that can be produced from biomass. The chemical method for xylitol synthesis is however expensive and energy intensive. In contrast, the biological route involving microbial cell factories offers a potential cost-effective alternative process. The bioprocess occurs under ambient conditions and makes use of biocatalysts which can be sourced from renewable carbon coming from a variety of cheap feedstocks classied as wastes. Result: In this study, biotransformation of xylose to xylitol was investigated using Yarrowia lipolytica an oleaginous yeast grown on glycerol/glucose screening of primary carbon source, media optimisation in shake ask, scale up in bioreactor and downstream processing of xylitol were carried out. -
Sugar Alcohols a Sugar Alcohol Is a Kind of Alcohol Prepared from Sugars
Sweeteners, Good, Bad, or Something even Worse. (Part 8) These are Low calorie sweeteners - not non-calorie sweeteners Sugar Alcohols A sugar alcohol is a kind of alcohol prepared from sugars. These organic compounds are a class of polyols, also called polyhydric alcohol, polyalcohol, or glycitol. They are white, water-soluble solids that occur naturally and are used widely in the food industry as thickeners and sweeteners. In commercial foodstuffs, sugar alcohols are commonly used in place of table sugar (sucrose), often in combination with high intensity artificial sweeteners to counter the low sweetness of the sugar alcohols. Unlike sugars, sugar alcohols do not contribute to the formation of tooth cavities. Common Sugar Alcohols Arabitol, Erythritol, Ethylene glycol, Fucitol, Galactitol, Glycerol, Hydrogenated Starch – Hydrolysate (HSH), Iditol, Inositol, Isomalt, Lactitol, Maltitol, Maltotetraitol, Maltotriitol, Mannitol, Methanol, Polyglycitol, Polydextrose, Ribitol, Sorbitol, Threitol, Volemitol, Xylitol, Of these, xylitol is perhaps the most popular due to its similarity to sucrose in visual appearance and sweetness. Sugar alcohols do not contribute to tooth decay. However, consumption of sugar alcohols does affect blood sugar levels, although less than that of "regular" sugar (sucrose). Sugar alcohols may also cause bloating and diarrhea when consumed in excessive amounts. Erythritol Also labeled as: Sugar alcohol Zerose ZSweet Erythritol is a sugar alcohol (or polyol) that has been approved for use as a food additive in the United States and throughout much of the world. It was discovered in 1848 by British chemist John Stenhouse. It occurs naturally in some fruits and fermented foods. At the industrial level, it is produced from glucose by fermentation with a yeast, Moniliella pollinis. -
Chapter 19 the Chemistry of Aldehydes and Ketones. Addition Reactions
Instructor Supplemental Solutions to Problems © 2010 Roberts and Company Publishers Chapter 19 The Chemistry of Aldehydes and Ketones. Addition Reactions Solutions to In-Text Problems 19.1 (b) (d) (e) (g) 19.2 (a) 2-Propanone (d) (E)-3-Ethoxy-2-propenal (f) 4,4-Dimethyl-2,5-cyclohexadienone 19.3 (b) 2-Cyclohexenone has a lower carbonyl stretching frequency because its two double bonds are conjugated. 19.4 (b) The compound is 2-butanone: (c) The high frequency of the carbonyl absorption suggests a strained ring. (See Eq. 19.4, text p. 897.) In fact, cyclobutanone matches the IR stretching frequency perfectly and the NMR fits as well: 19.6 The structure and CMR assignments of 2-ethylbutanal are shown below. The two methyl groups are chemically equivalent, and the two methylene groups are chemically equivalent; all carbons with different CMR chemical shifts are chemically nonequivalent. INSTRUCTOR SUPPLEMENTAL SOLUTIONS TO PROBLEMS • CHAPTER 19 2 19.7 (a) The double bonds in 2-cyclohexenone are conjugated, but the double bonds in 3-cyclohexenone are not. Consequently, 2-cyclohexenone has the UV spectrum with the greater lmax. 19.9 Compound A, vanillin, should have a p T p* absorption at a greater lmax when dissolved in NaOH solution because the resulting phenolate can delocalize into the carboxaldehyde group; the resulting phenolate from compound B, isovanillin, on the other hand, can only delocalize in the aromatic ring. 19.11 The mass spectrum of 2-heptanone should have major peaks at m/z = 43 (from a-cleavage), 71 (from inductive cleavage), and 58 (from McLafferty rearrangement). -
Temporary Compounding of Certain Alcohol-Based Hand Sanitizer Products During the Public Health Emergency Immediately in Effect Guidance for Industry
Policy for Temporary Compounding of Certain Alcohol-Based Hand Sanitizer Products During the Public Health Emergency Immediately in Effect Guidance for Industry FDA is issuing this guidance for immediate implementation in accordance with 21 CFR 10.115(g)(2). Comments may be submitted at any time for Agency consideration. Submit written comments to the Dockets Management Staff (HFA-305), Food and Drug Administration, 5630 Fishers Lane, Rm. 1061, Rockville, MD 20852. Submit electronic comments to https://www.regulations.gov. All comments should be identified with the docket number listed in the notice of availability that publishes in the Federal Register. For questions regarding this document, contact FDA’s human drug compounding team (CDER) at [email protected]. March 2020 Updated February 10, 2021 Compounding Contains Nonbinding Recommendations Policy for Temporary Compounding of Certain Alcohol-Based Hand Sanitizer Products During the Public Health Emergency Immediately in Effect Guidance for Industry Additional copies are available from: Office of Communications, Division of Drug Information Center for Drug Evaluation and Research Food and Drug Administration 10001 New Hampshire Ave., Hillandale Bldg., 4th Floor Silver Spring, MD 20993-0002 Phone: 855-543-3784 or 301-796-3400; Fax: 301-431-6353 Email: [email protected] https://www.fda.gov/drugs/guidance-compliance-regulatory-information/guidances-drugs U.S. Department of Health and Human Services Food and Drug Administration Center for Drug Evaluation and Research -
Toward the Sustainable Synthesis of Propanols from Renewable Glycerol Over Moo3-Al2o3 Supported Palladium Catalysts
catalysts Article Toward the Sustainable Synthesis of Propanols from Renewable Glycerol over MoO3-Al2O3 Supported Palladium Catalysts Shanthi Priya Samudrala * and Sankar Bhattacharya Department of Chemical Engineering, Faculty of Engineering, Monash University, Melbourne 3800, Australia; [email protected] * Correspondence: [email protected]; Tel.: +61-3-9905-8162 Received: 17 August 2018; Accepted: 6 September 2018; Published: 9 September 2018 Abstract: The catalytic conversion of glycerol to value-added propanols is a promising synthetic route that holds the potential to overcome the glycerol oversupply from the biodiesel industry. In this study, selective hydrogenolysis of 10 wt% aqueous bio-glycerol to 1-propanol and 2-propanol was performed in the vapor phase, fixed-bed reactor by using environmentally friendly bifunctional Pd/MoO3-Al2O3 catalysts prepared by wetness impregnation method. The physicochemical properties of these catalysts were derived from various techniques such as X-ray diffraction, 27 NH3-temperature programmed desorption, scanning electron microscopy, Al NMR spectroscopy, surface area analysis, and thermogravimetric analysis. The catalytic activity results depicted that a high catalytic activity (>80%) with very high selectivity (>90%) to 1-propanol and 2-propanol was obtained over all the catalysts evaluated in a continuously fed, fixed-bed reactor. However, among all others, 2 wt% Pd/MoO3-Al2O3 catalyst was the most active and selective to propanols. The synergic interaction between the palladium and MoO3 on Al2O3 support and high strength weak to moderate acid sites of the catalyst were solely responsible for the high catalytic activity. The maximum glycerol conversion of 88.4% with 91.3% selectivity to propanols was achieved at an optimum reaction condition of 210 ◦C and 1 bar pressure after 3 h of glycerol hydrogenolysis reaction. -
Compounding Alcohol-Based Hand Sanitizer During COVID-19 Pandemic
Compounding Alcohol-Based Hand Sanitizer During COVID-19 Pandemic Updated: March 25, 2020 This document is for informational purposes only for healthcare practitioners and scientific professionals, and is intended to address shortages of alcohol-based hand sanitizers associated with the COVID-19 pandemic. This does not reflect the Compounding Expert Committee’s opinions on future development or revisions to official text of the USP-NF. USP is actively monitoring the evolving situation and will update this document accordingly. Parties relying on the information in this document bear independent responsibility for awareness of, and compliance with, any applicable federal, state, or local laws and requirements. Summary of updates: ` March 25, 2020. Recommendations were added to respond to stakeholder questions about substitutions in light of shortages of ingredients. Formulation 3 was revised due to inherent variability in the raw materials and volatility to ensure that the isopropyl alcohol concentration exceeds the recommendations by the Centers for Disease Control (CDC). Background and Introduction In light of the rapidly evolving COVID-19 pandemic, there is an expected shortage of alcohol-based hand sanitizers. The CDC recommends washing hands with soap and water whenever possible because handwashing reduces the amounts of all types of germs and chemicals on hands.1 If soap and water are not available, using a hand sanitizer with a final concentration of at least 60% ethanol or 70% isopropyl alcohol inactivates viruses that are genetically -
USP Alcohol-Based Hand Sanitizer Infographic
Compounding Alcohol-Based Demand for alcohol-based hand sanitizers has surpassed its supply due to the rapidly evolving Hand Sanitizer During COVID-19 pandemic. In response, the U.S. Pharmacopeia has provided an informational resource COVID-19 Pandemic document on compounding hand sanitizer to help address the shortages. Please visit usp.org/compounding for more info.* Ethanol 1,2 Isopropyl Isopropyl 96% Alcohol 1 99% Alcohol 1 91% 8333 mL 7576 mL 8242 mL Hydrogen Hydrogen Hydrogen peroxide 3% peroxide 3% peroxide 3% 417 mL 417 mL 417 mL Glycerol 3 Glycerol 3 Glycerol 3 98% 98% 98% 145 mL 75 mL 75 mL Water 4 Water 4 Water 4 suicient a suicient a suicient quantity to quantity to quantity to make make make 10,000 mL 10,000 mL 10,000 mL Formulation 1 Formulation 2 Formulation 3 Formulation 1 When the concentration of alcohol in the starting ingredient is not exact, 2 It is recommended to use denatured ethanol instead of nondenatured ethanol 3 Glycerin and glycerol are synonymous 4 Water may be distilled, cold boiled follow the calculation on the USP hand sanitizer resource document to because there have been reports of adverse events, including deaths, from and may be interchanged. Glycerin and potable, reverse osmosis, or filtered. ensure a final concentration of at least 80% ethanol or 75% isopropyl alcohol. unintentional ingestion of hand sanitizer, particularly in young children. If it is glycerol are added as humectants. not denatured, package in a child-resistant container. Packaging and Storage: Labeling: Label it to state for external Beyond-Use Date: NMT 30 days Package in well-closed, use only, the percentage of active after the date on which it was suitable containers and store at ingredient (i.e., ethanol, isopropyl compounded when stored at controlled room temperature. -
The Role of Glycerol and Its Derivatives in the Biochemistry of Living Organisms, and Their Prebiotic Origin and Significance in the Evolution of Life
catalysts Review The Role of Glycerol and Its Derivatives in the Biochemistry of Living Organisms, and Their Prebiotic Origin and Significance in the Evolution of Life Maheen Gull * and Matthew A. Pasek School of Geosciences, University of South Florida, 4202 E Fowler Ave., NES 204, Tampa, FL 33620, USA; [email protected] * Correspondence: [email protected]; Tel.: +1-813-974-8979 Abstract: The emergence and evolution of prebiotic biomolecules on the early Earth remain a question that is considered crucial to understanding the chemistry of the origin of life. Amongst prebiotic molecules, glycerol is significant due to its ubiquity in biochemistry. In this review, we discuss the significance of glycerol and its various derivatives in biochemistry, their plausible roles in the origin and evolution of early cell membranes, and significance in the biochemistry of extremophiles, followed by their prebiotic origin on the early Earth and associated catalytic processes that led to the origin of these compounds. We also discuss various scenarios for the prebiotic syntheses of glycerol and its derivates and evaluate these to determine their relevance to early Earth biochemistry and geochemistry, and recapitulate the utilization of various minerals (including clays), condensation agents, and solvents that could have led to the successful prebiotic genesis of these biomolecules. Furthermore, important prebiotic events such as meteoritic delivery and prebiotic synthesis reactions under astrophysical conditions are also discussed. Finally, we have also highlighted some novel features of glycerol, including glycerol nucleic acid (GNA), in the origin and evolution of the life. Citation: Gull, M.; Pasek, M.A. The Keywords: glycerol; phosphorylation; catalysis; prebiotic syntheses; origin of life; evolution of cell Role of Glycerol and Its Derivatives in membranes; deep eutectic solvents; extremophiles; glycerol biochemistry; phospholipids; early Earth the Biochemistry of Living Organisms, and Their Prebiotic Origin and Significance in the Evolution of Life. -
Optimum Volume Ratio of Sorbitol and Glycerol As Plasticizer on Bioplastic from Tapioca Starch
OPTIMUM VOLUME RATIO OF SORBITOL AND GLYCEROL AS PLASTICIZER ON BIOPLASTIC FROM TAPIOCA STARCH Compiled as one of the requirements of completing the bachelor degree in the study program of chemical engineering By: YOCHI ASIANOV D500154004 CHEMICAL ENGINEERING DEPARTMENT ENGINEERING FACULTY UNIVERSITAS MUHAMMADIYAH SURAKARTA 2019 i APPROVAL LETTER OPTIMUM VOLUME RATIO OF SORBITOL AND GLYCEROL AS PLASTICIZER ON BIOPLASTIC FROM TAPIOCA STARCH SCIENCE PUBLICATION By: YOCHI ASIANOV D500154004 Has been reviewed and approved by: Supervisor, Hamid Abdillah, S.T., M.T NIK. 894 i ACCEPTANCE LETTER OPTIMUM VOLUME RATIO OF SORBITOL AND GLYCEROL AS PLASTICIZER ON BIOPLASTIC FROM TAPIOCA STARCH By: YOCHI ASIANOV D500154004 It has been approved by the examiners Faculty of Engineering Universitas Muhammadiyah Surakarta On, July 20th 2019 On, April 2019 And declared eligible Examiners: 1. Hamid Abdillah, S.T., M.T ( ) (Chairman of examiners) 2. Emi Erawati, S.T., M.Eng ( ) (Examiner I) 3. Herry Purnama, S.T., M.T., Ph.D ( ) (Examiner II) Dean, Ir. Sri Sunarjono, S.T., M.T., Ph.D NIK. 682 ii PRONOUNCEMENT Herewith, I testify that in this publication article there is no plagiarism of the previous literary work which has been raised to obtain bachelor degree of a university, nor there are opinions or masterpieces which have been written or published by others, except those in which the writing are reffered in manuscript and mentioned in literary reviews and bibliography. If later, the result of this study are proven as plagiarism, I will be fully responsible and willing to accept sanction in accordance with applicable regulation. Surakarta, July 20th 2019 Writer, Yochi Asianov D500154004 iii OPTIMUM VOLUME RATIO OF SORBITOL AND GLYCEROL AS PLASTICIZER ON BIOPLASTIC FROM TAPIOCA STARCH Abstrak Permintaan plastik di seluruh dunia terus meningkat, hampir setiap produk menggunakan plastik sebagai kemasan.