Cosmology at the Turning Point of Relativity Revolution. the Debates During the 1920’S on the “De Sitter Effect”
Total Page:16
File Type:pdf, Size:1020Kb
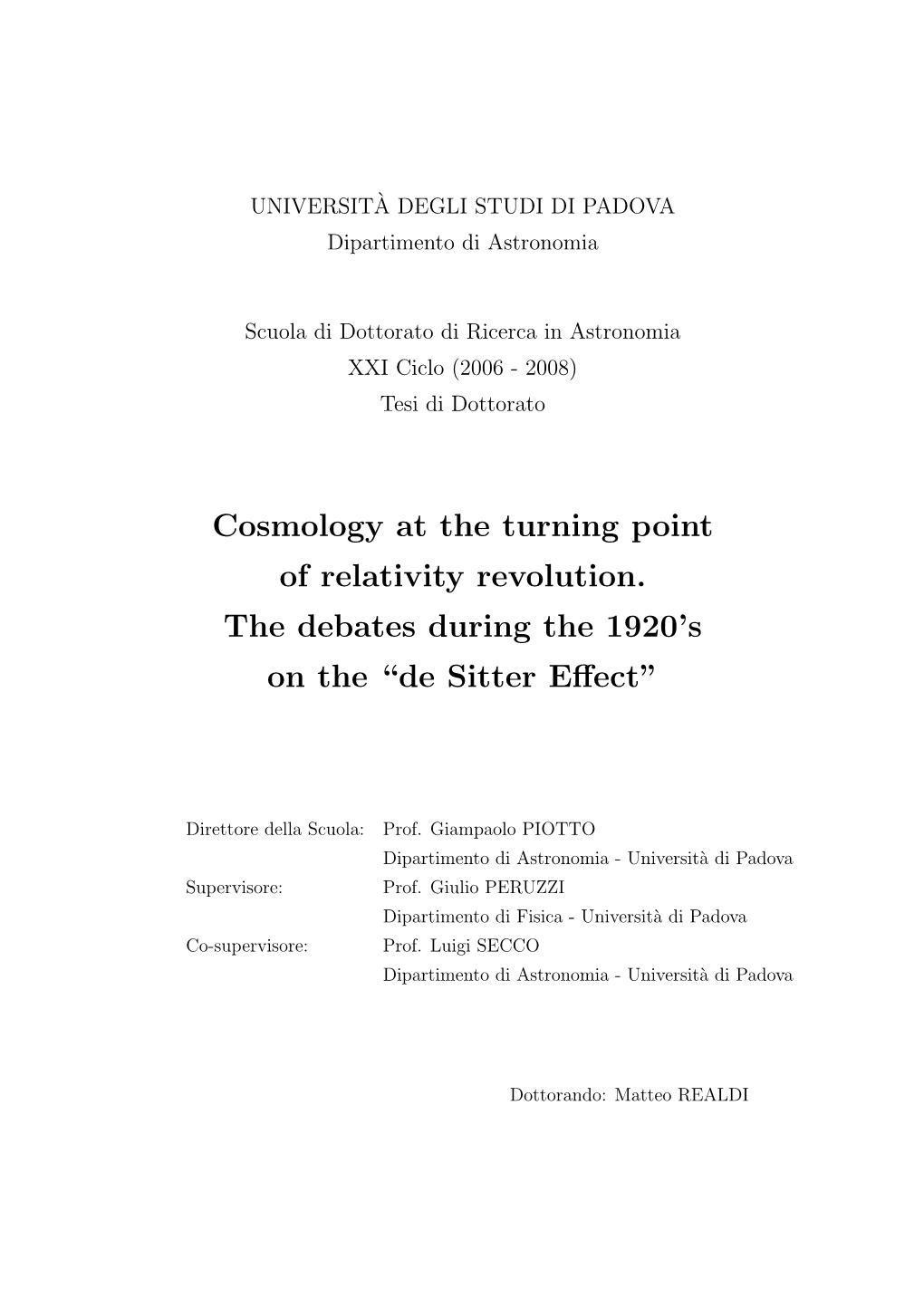
Load more
Recommended publications
-
Birth of De Sitter Universe from a Time Crystal Universe
PHYSICAL REVIEW D 100, 123531 (2019) Birth of de Sitter universe from a time crystal universe † Daisuke Yoshida * and Jiro Soda Department of Physics, Kobe University, Kobe 657-8501, Japan (Received 30 September 2019; published 18 December 2019) We show that a simple subclass of Horndeski theory can describe a time crystal universe. The time crystal universe can be regarded as a baby universe nucleated from a flat space, which is mediated by an extension of Giddings-Strominger instanton in a 2-form theory dual to the Horndeski theory. Remarkably, when a cosmological constant is included, de Sitter universe can be created by tunneling from the time crystal universe. It gives rise to a past completion of an inflationary universe. DOI: 10.1103/PhysRevD.100.123531 I. INTRODUCTION Euler-Lagrange equations of motion contain up to second order derivatives. Actually, bouncing cosmology in the Inflation has succeeded in explaining current observa- Horndeski theory has been intensively investigated so far tions of the large scale structure of the Universe [1,2]. [23–29]. These analyses show the presence of gradient However, inflation has a past boundary [3], which could be instability [30,31] and finally no-go theorem for stable a true initial singularity or just a coordinate singularity bouncing cosmology in Horndeski theory was found for the depending on how much the Universe deviates from the spatially flat universe [32]. However, it was shown that the exact de Sitter [4], or depending on the topology of the no-go theorem does not hold when the spatial curvature is Universe [5]. -
Expanding Space, Quasars and St. Augustine's Fireworks
Universe 2015, 1, 307-356; doi:10.3390/universe1030307 OPEN ACCESS universe ISSN 2218-1997 www.mdpi.com/journal/universe Article Expanding Space, Quasars and St. Augustine’s Fireworks Olga I. Chashchina 1;2 and Zurab K. Silagadze 2;3;* 1 École Polytechnique, 91128 Palaiseau, France; E-Mail: [email protected] 2 Department of physics, Novosibirsk State University, Novosibirsk 630 090, Russia 3 Budker Institute of Nuclear Physics SB RAS and Novosibirsk State University, Novosibirsk 630 090, Russia * Author to whom correspondence should be addressed; E-Mail: [email protected]. Academic Editors: Lorenzo Iorio and Elias C. Vagenas Received: 5 May 2015 / Accepted: 14 September 2015 / Published: 1 October 2015 Abstract: An attempt is made to explain time non-dilation allegedly observed in quasar light curves. The explanation is based on the assumption that quasar black holes are, in some sense, foreign for our Friedmann-Robertson-Walker universe and do not participate in the Hubble flow. Although at first sight such a weird explanation requires unreasonably fine-tuned Big Bang initial conditions, we find a natural justification for it using the Milne cosmological model as an inspiration. Keywords: quasar light curves; expanding space; Milne cosmological model; Hubble flow; St. Augustine’s objects PACS classifications: 98.80.-k, 98.54.-h You’d think capricious Hebe, feeding the eagle of Zeus, had raised a thunder-foaming goblet, unable to restrain her mirth, and tipped it on the earth. F.I.Tyutchev. A Spring Storm, 1828. Translated by F.Jude [1]. 1. Introduction “Quasar light curves do not show the effects of time dilation”—this result of the paper [2] seems incredible. -
3 the Friedmann-Robertson-Walker Metric
3 The Friedmann-Robertson-Walker metric 3.1 Three dimensions The most general isotropic and homogeneous metric in three dimensions is similar to the two dimensional result of eq. (43): dr2 ds2 = a2 + r2dΩ2 ; dΩ2 = dθ2 + sin2 θdφ2 ; k = 0; 1 : (46) 1 kr2 − The angles φ and θ are the usual azimuthal and polar angles of spherical coordinates, with θ [0; π], φ [0; 2π). As before, the parameter k can take on three different values: for k = 0, 2 2 the above line element describes ordinary flat space in spherical coordinates; k = 1 yields the metric for S , with constant positive curvature, while k = 1 is AdS and has constant 3 − 3 negative curvature. As in the two dimensional case, the change of variables r = sin χ (k = 1) or r = sinh χ (k = 1) makes the global nature of these manifolds more apparent. For example, − for the k = 1 case, after defining r = sin χ, the line element becomes ds2 = a2 dχ2 + sin2 χdθ2 + sin2 χ sin2 θdφ2 : (47) This is equivalent to writing ds2 = dX2 + dY 2 + dZ2 + dW 2 ; (48) where X = a sin χ sin θ cos φ ; Y = a sin χ sin θ sin φ ; Z = a sin χ cos θ ; W = a cos χ ; (49) which satisfy X2 + Y 2 + Z2 + W 2 = a2. So we see that the k = 1 metric corresponds to a 3-sphere of radius a embedded in 4-dimensional Euclidean space. One also sees a problem with the r = sin χ coordinate: it does not cover the whole sphere. -
The Son of Lamoraal Ulbo De Sitter, a Judge, and Catharine Theodore Wilhelmine Bertling
558 BIOGRAPHIES v.i WiLLEM DE SITTER viT 1872-1934 De Sitter was bom on 6 May 1872 in Sneek (province of Friesland), the son of Lamoraal Ulbo de Sitter, a judge, and Catharine Theodore Wilhelmine Bertling. His father became presiding judge of the court in Arnhem, and that is where De Sitter attended gymna sium. At the University of Groniiigen he first studied mathematics and physics and then switched to astronomy under Jacobus Kapteyn. De Sitter spent two years observing and studying under David Gill at the Cape Obsen'atory, the obseivatory with which Kapteyn was co operating on the Cape Photographic Durchmusterung. De Sitter participated in the program to make precise measurements of the positions of the Galilean moons of Jupiter, using a heliometer. In 1901 he received his doctorate under Kapteyn on a dissertation on Jupiter's satellites: Discussion of Heliometer Observations of Jupiter's Satel lites. De Sitter remained at Groningen as an assistant to Kapteyn in the astronomical laboratory, until 1909, when he was appointed to the chair of astronomy at the University of Leiden. In 1919 he be came director of the Leiden Observatory. He remained in these posts until his death in 1934. De Sitter's work was highly mathematical. With his work on Jupi ter's satellites, De Sitter pursued the new methods of celestial me chanics of Poincare and Tisserand. His earlier heliometer meas urements were later supplemented by photographic measurements made at the Cape, Johannesburg, Pulkowa, Greenwich, and Leiden. De Sitter's final results on this subject were published as 'New Math ematical Theory of Jupiter's Satellites' in 1925. -
An Analysis of Gravitational Redshift from Rotating Body
An analysis of gravitational redshift from rotating body Anuj Kumar Dubey∗ and A K Sen† Department of Physics, Assam University, Silchar-788011, Assam, India. (Dated: July 29, 2021) Gravitational redshift is generally calculated without considering the rotation of a body. Neglect- ing the rotation, the geometry of space time can be described by using the spherically symmetric Schwarzschild geometry. Rotation has great effect on general relativity, which gives new challenges on gravitational redshift. When rotation is taken into consideration spherical symmetry is lost and off diagonal terms appear in the metric. The geometry of space time can be then described by using the solutions of Kerr family. In the present paper we discuss the gravitational redshift for rotating body by using Kerr metric. The numerical calculations has been done under Newtonian approximation of angular momentum. It has been found that the value of gravitational redshift is influenced by the direction of spin of central body and also on the position (latitude) on the central body at which the photon is emitted. The variation of gravitational redshift from equatorial to non - equatorial region has been calculated and its implications are discussed in detail. I. INTRODUCTION from principle of equivalence. Snider in 1972 has mea- sured the redshift of the solar potassium absorption line General relativity is not only relativistic theory of grav- at 7699 A˚ by using an atomic - beam resonance - scat- itation proposed by Einstein, but it is the simplest theory tering technique [5]. Krisher et al. in 1993 had measured that is consistent with experimental data. Predictions of the gravitational redshift of Sun [6]. -
On Asymptotically De Sitter Space: Entropy & Moduli Dynamics
On Asymptotically de Sitter Space: Entropy & Moduli Dynamics To Appear... [1206.nnnn] Mahdi Torabian International Centre for Theoretical Physics, Trieste String Phenomenology Workshop 2012 Isaac Newton Institute , CAMBRIDGE 1 Tuesday, June 26, 12 WMAP 7-year Cosmological Interpretation 3 TABLE 1 The state of Summarythe ofart the cosmological of Observational parameters of ΛCDM modela Cosmology b c Class Parameter WMAP 7-year ML WMAP+BAO+H0 ML WMAP 7-year Mean WMAP+BAO+H0 Mean 2 +0.056 Primary 100Ωbh 2.227 2.253 2.249−0.057 2.255 ± 0.054 2 Ωch 0.1116 0.1122 0.1120 ± 0.0056 0.1126 ± 0.0036 +0.030 ΩΛ 0.729 0.728 0.727−0.029 0.725 ± 0.016 ns 0.966 0.967 0.967 ± 0.014 0.968 ± 0.012 τ 0.085 0.085 0.088 ± 0.015 0.088 ± 0.014 2 d −9 −9 −9 −9 ∆R(k0) 2.42 × 10 2.42 × 10 (2.43 ± 0.11) × 10 (2.430 ± 0.091) × 10 +0.030 Derived σ8 0.809 0.810 0.811−0.031 0.816 ± 0.024 H0 70.3km/s/Mpc 70.4km/s/Mpc 70.4 ± 2.5km/s/Mpc 70.2 ± 1.4km/s/Mpc Ωb 0.0451 0.0455 0.0455 ± 0.0028 0.0458 ± 0.0016 Ωc 0.226 0.226 0.228 ± 0.027 0.229 ± 0.015 2 +0.0056 Ωmh 0.1338 0.1347 0.1345−0.0055 0.1352 ± 0.0036 e zreion 10.4 10.3 10.6 ± 1.210.6 ± 1.2 f t0 13.79 Gyr 13.76 Gyr 13.77 ± 0.13 Gyr 13.76 ± 0.11 Gyr a The parameters listed here are derived using the RECFAST 1.5 and version 4.1 of the WMAP[WMAP-7likelihood 1001.4538] code. -
Aspects of Spatially Homogeneous and Isotropic Cosmology
Faculty of Technology and Science Department of Physics and Electrical Engineering Mikael Isaksson Aspects of Spatially Homogeneous and Isotropic Cosmology Degree Project of 15 credit points Physics Program Date/Term: 02-04-11 Supervisor: Prof. Claes Uggla Examiner: Prof. Jürgen Fuchs Karlstads universitet 651 88 Karlstad Tfn 054-700 10 00 Fax 054-700 14 60 [email protected] www.kau.se Abstract In this thesis, after a general introduction, we first review some differential geom- etry to provide the mathematical background needed to derive the key equations in cosmology. Then we consider the Robertson-Walker geometry and its relation- ship to cosmography, i.e., how one makes measurements in cosmology. We finally connect the Robertson-Walker geometry to Einstein's field equation to obtain so- called cosmological Friedmann-Lema^ıtre models. These models are subsequently studied by means of potential diagrams. 1 CONTENTS CONTENTS Contents 1 Introduction 3 2 Differential geometry prerequisites 8 3 Cosmography 13 3.1 Robertson-Walker geometry . 13 3.2 Concepts and measurements in cosmography . 18 4 Friedmann-Lema^ıtre dynamics 30 5 Bibliography 42 2 1 INTRODUCTION 1 Introduction Cosmology comes from the Greek word kosmos, `universe' and logia, `study', and is the study of the large-scale structure, origin, and evolution of the universe, that is, of the universe taken as a whole [1]. Even though the word cosmology is relatively recent (first used in 1730 in Christian Wolff's Cosmologia Generalis), the study of the universe has a long history involving science, philosophy, eso- tericism, and religion. Cosmologies in their earliest form were concerned with, what is now known as celestial mechanics (the study of the heavens). -
Surface Curvature-Quantized Energy and Forcefield in Geometrochemical Physics
Title: Surface curvature-quantized energy and forcefield in geometrochemical physics Author: Z. R. Tian, Univ. of Arkansas, Fayetteville, AR 72701, USA, (Email) [email protected]. Text: Most recently, simple-shape particles’ surface area-to-volume ratio has been quantized into a geometro-wavenumber (Geo), or geometro-energy (EGeo) hcGeo, to quantitatively predict and compare nanoparticles (NPs), ions, and atoms geometry- quantized properties1,2. These properties range widely, from atoms’ electronegativity (EN) and ionization potentials to NPs’ bonding energy, atomistic nature, chemical potential of formation, redox potential, surface adsorbates’ stability, and surface defects’ reactivity. For countless irregular- or complex-shaped molecules, clusters, and NPs whose Geo values are uneasy to calculate, however, the EGeo application seems limited. This work has introduced smaller surfaces’ higher curvature-quantized energy and forcefield for linking quantum mechanics, thermodynamics, electromagnetics, and Newton’s mechanics with Einstein’s general relativity. This helps quantize the gravity, gravity-counterbalancing levity, and Einstein’s spacetime, geometrize Heisenberg’s Uncertainty, and support many known theories consistently for unifying naturally the energies and forcefields in physics, chemistry, and biology. Firstly, let’s quantize shaper corners’ 1.24 (keVnm). Indeed, smaller atoms’ higher and edges’ greater surface curvature (1/r) into EN and smaller 0-dimensional (0D) NPs’ a spacetime wavenumber (ST), i.e. Spacetime lower melting point (Tm) and higher (i.e. Energy (EST) = hc(ST) = hc(1/r) (see the Fig. more blue-shifted) optical bandgap (EBG) (see 3 below), where the r = particle radius, h = the Supplementary Table S1)3-9 are linearly Planck constant, c = speed of light, and hc governed by their greater (1/r) i.e. -
Gravitational Redshift/Blueshift of Light Emitted by Geodesic
Eur. Phys. J. C (2021) 81:147 https://doi.org/10.1140/epjc/s10052-021-08911-5 Regular Article - Theoretical Physics Gravitational redshift/blueshift of light emitted by geodesic test particles, frame-dragging and pericentre-shift effects, in the Kerr–Newman–de Sitter and Kerr–Newman black hole geometries G. V. Kraniotisa Section of Theoretical Physics, Physics Department, University of Ioannina, 451 10 Ioannina, Greece Received: 22 January 2020 / Accepted: 22 January 2021 / Published online: 11 February 2021 © The Author(s) 2021 Abstract We investigate the redshift and blueshift of light 1 Introduction emitted by timelike geodesic particles in orbits around a Kerr–Newman–(anti) de Sitter (KN(a)dS) black hole. Specif- General relativity (GR) [1] has triumphed all experimental ically we compute the redshift and blueshift of photons that tests so far which cover a wide range of field strengths and are emitted by geodesic massive particles and travel along physical scales that include: those in large scale cosmology null geodesics towards a distant observer-located at a finite [2–4], the prediction of solar system effects like the perihe- distance from the KN(a)dS black hole. For this purpose lion precession of Mercury with a very high precision [1,5], we use the killing-vector formalism and the associated first the recent discovery of gravitational waves in Nature [6–10], integrals-constants of motion. We consider in detail stable as well as the observation of the shadow of the M87 black timelike equatorial circular orbits of stars and express their hole [11], see also [12]. corresponding redshift/blueshift in terms of the metric physi- The orbits of short period stars in the central arcsecond cal black hole parameters (angular momentum per unit mass, (S-stars) of the Milky Way Galaxy provide the best current mass, electric charge and the cosmological constant) and the evidence for the existence of supermassive black holes, in orbital radii of both the emitter star and the distant observer. -
Galaxy Formation Within the Classical Big Bang Cosmology
Galaxy formation within the classical Big Bang Cosmology Bernd Vollmer CDS, Observatoire astronomique de Strasbourg Outline • some basics of astronomy small structure • galaxies, AGNs, and quasars • from galaxies to the large scale structure of the universe large • the theory of cosmology structure • measuring cosmological parameters • structure formation • galaxy formation in the cosmological framework • open questions small structure The architecture of the universe • Earth (~10-9 ly) • solar system (~6 10-4 ly) • nearby stars (> 5 ly) • Milky Way (~6 104 ly) • Galaxies (>2 106 ly) • large scale structure (> 50 106 ly) How can we investigate the universe Astronomical objects emit elctromagnetic waves which we can use to study them. BUT The earth’s atmosphere blocks a part of the electromagnetic spectrum. need for satellites Back body radiation • Opaque isolated body at a constant temperature Wien’s law: sun/star (5500 K): 0.5 µm visible human being (310 K): 9 µm infrared molecular cloud (15 K): 200 µm radio The structure of an atom Components: nucleus (protons, neutrons) + electrons Bohr’s model: the electrons orbit around the nucleus, the orbits are discrete Bohr’s model is too simplistic quantum mechanical description The emission of an astronomical object has 2 components: (i) continuum emission + (ii) line emission Stellar spectra Observing in colors Usage of filters (Johnson): U (UV), B (blue), V (visible), R (red), I (infrared) The Doppler effect • Is the apparent change in frequency and wavelength of a wave which is emitted by -
A Stellar Flare-Coronal Mass Ejection Event Revealed by X-Ray Plasma Motions
A stellar flare-coronal mass ejection event revealed by X-ray plasma motions C. Argiroffi1,2⋆, F. Reale1,2, J. J. Drake3, A. Ciaravella2, P. Testa3, R. Bonito2, M. Miceli1,2, S. Orlando2, and G. Peres1,2 1 University of Palermo, Department of Physics and Chemistry, Piazza del Parlamento 1, 90134, Palermo, Italy. 2 INAF - Osservatorio Astronomico di Palermo, Piazza del Parlamento 1, 90134, Palermo, Italy. 3 Smithsonian Astrophysical Observatory, MS-3, 60 Garden Street, Cambridge, MA 02138, USA. ⋆ costanza.argiroffi@unipa.it May 28, 2019 Coronal mass ejections (CMEs), often associ- transported along the magnetic field lines and heats ated with flares 1,2,3, are the most powerful mag- the underlying chromosphere, that expands upward netic phenomena occurring on the Sun. Stars at hundreds of kms−1, filling the overlying magnetic show magnetic activity levels up to 104 times structure (flare rising phase). Then this plasma gradu- higher 4, and CME effects on stellar physics and ally cools down radiatively and conductively (flare de- circumstellar environments are predicted to be cay). The flare magnetic drivers often cause also large- significant 5,6,7,8,9. However, stellar CMEs re- scale expulsions of previously confined plasma, CMEs, main observationally unexplored. Using time- that carry away large amounts of mass and energy. resolved high-resolution X-ray spectroscopy of a Solar observations demonstrate that CME occurrence, stellar flare on the active star HR 9024 observed mass, and kinetic energy increase with increasing flare with Chandra/HETGS, we distinctly detected energy 1,2, corroborating the flare-CME link. Doppler shifts in S xvi, Si xiv, and Mg xii lines Active stars have stronger magnetic fields, higher that indicate upward and downward motions of flare energies, hotter and denser coronal plasma 12. -
An Interpretation of Milne Cosmology
An Interpretation of Milne Cosmology Alasdair Macleod University of the Highlands and Islands Lews Castle College Stornoway Isle of Lewis HS2 0XR UK [email protected] Abstract The cosmological concordance model is consistent with all available observational data, including the apparent distance and redshift relationship for distant supernovae, but it is curious how the Milne cosmological model is able to make predictions that are similar to this preferred General Relativistic model. Milne’s cosmological model is based solely on Special Relativity and presumes a completely incompatible redshift mechanism; how then can the predictions be even remotely close to observational data? The puzzle is usually resolved by subsuming the Milne Cosmological model into General Relativistic cosmology as the special case of an empty Universe. This explanation may have to be reassessed with the finding that spacetime is approximately flat because of inflation, whereupon the projection of cosmological events onto the observer’s Minkowski spacetime must always be kinematically consistent with Special Relativity, although the specific dynamics of the underlying General Relativistic model can give rise to virtual forces in order to maintain consistency between the observation and model frames. I. INTRODUCTION argument is that a clear distinction must be made between models, which purport to explain structure and causes (the Edwin Hubble’s discovery in the 1920’s that light from extra- ‘Why?’), and observational frames which simply impart galactic nebulae is redshifted in linear proportion to apparent consistency and causality on observation (the ‘How?’). distance was quickly associated with General Relativity (GR), and explained by the model of a closed finite Universe curved However, it can also be argued this approach does not actually under its own gravity and expanding at a rate constrained by the explain the similarity in the predictive power of Milne enclosed mass.