On the Characteristics and Evolution of Dynamically Excited Trans-Neptunian Objects
Total Page:16
File Type:pdf, Size:1020Kb
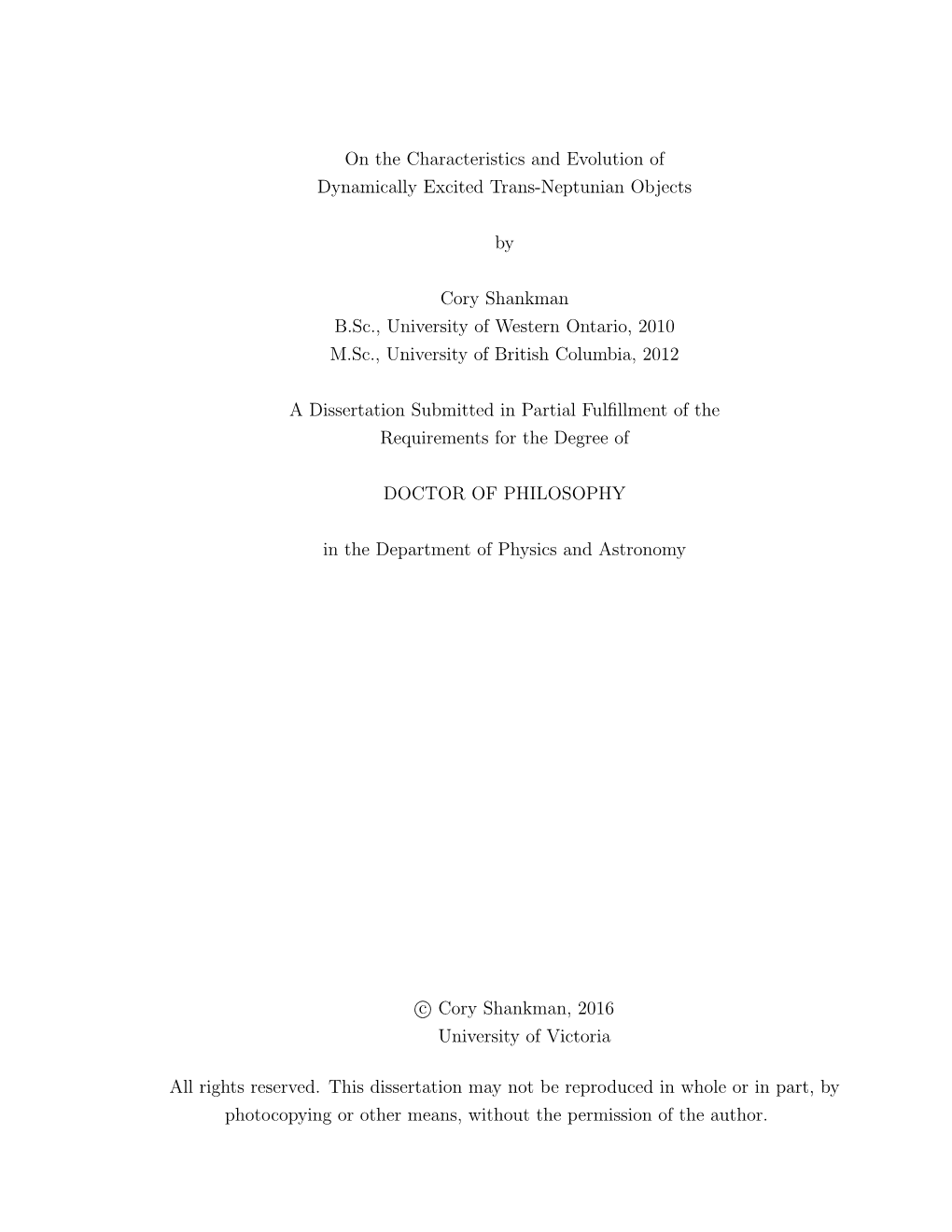
Load more
Recommended publications
-
Astro2020 Science White Paper Modeling Debris Disk Evolution
Astro2020 Science White Paper Modeling Debris Disk Evolution Thematic Areas Planetary Systems Formation and Evolution of Compact Objects Star and Planet Formation Cosmology and Fundamental Physics Galaxy Evolution Resolved Stellar Populations and their Environments Stars and Stellar Evolution Multi-Messenger Astronomy and Astrophysics Principal Author András Gáspár Steward Observatory, The University of Arizona [email protected] 1-(520)-621-1797 Co-Authors Dániel Apai, Jean-Charles Augereau, Nicholas P. Ballering, Charles A. Beichman, Anthony Boc- caletti, Mark Booth, Brendan P. Bowler, Geoffrey Bryden, Christine H. Chen, Thayne Currie, William C. Danchi, John Debes, Denis Defrère, Steve Ertel, Alan P. Jackson, Paul G. Kalas, Grant M. Kennedy, Matthew A. Kenworthy, Jinyoung Serena Kim, Florian Kirchschlager, Quentin Kral, Sebastiaan Krijt, Alexander V. Krivov, Marc J. Kuchner, Jarron M. Leisenring, Torsten Löhne, Wladimir Lyra, Meredith A. MacGregor, Luca Matrà, Dimitri Mawet, Bertrand Mennes- son, Tiffany Meshkat, Amaya Moro-Martín, Erika R. Nesvold, George H. Rieke, Aki Roberge, Glenn Schneider, Andrew Shannon, Christopher C. Stark, Kate Y. L. Su, Philippe Thébault, David J. Wilner, Mark C. Wyatt, Marie Ygouf, Andrew N. Youdin Co-Signers Virginie Faramaz, Kevin Flaherty, Hannah Jang-Condell, Jérémy Lebreton, Sebastián Marino, Jonathan P. Marshall, Rafael Millan-Gabet, Peter Plavchan, Luisa M. Rebull, Jacob White Abstract Understanding the formation, evolution, diversity, and architectures of planetary systems requires detailed knowledge of all of their components. The past decade has shown a remarkable increase in the number of known exoplanets and debris disks, i.e., populations of circumstellar planetes- imals and dust roughly analogous to our solar system’s Kuiper belt, asteroid belt, and zodiacal dust. -
The Origin of the High-Inclination Neptune Trojan 2005 TN53 J
A&A 464, 775–778 (2007) Astronomy DOI: 10.1051/0004-6361:20066297 & c ESO 2007 Astrophysics The origin of the high-inclination Neptune Trojan 2005 TN53 J. Li, L.-Y. Zhou, and Y.-S. Sun Department of Astronomy, Nanjing University, Nanjing 210093, PR China e-mail: [email protected] Received 25 August 2006 / Accepted 20 November 2006 ABSTRACT Aims. We explore the formation and evolution of the highly inclined orbit of Neptune Trojan 2005 TN53. Methods. With numerical simulations, we investigated a possible mechanism for the origin of the high-inclination Neptune Trojans as captured into the Trojan-type orbits by an initially eccentric Neptune during its eccentricity damping and rapid inward migration, then migrating to the present locations locked in Neptune’s 1:1 mean motion resonance. ◦ Results. Two 2005 TN53-type Trojans out of our 2000 test particles were produced with inclinations above 20 , moving on tadpole orbits librating around Neptune’s leading Lagrange point. Key words. methods: numerical – celestial mechanics – minor planets, asteroids – solar system: formation 1. Introduction Table 1. Orbital elements of 2005 TN53 in heliocentric frame referred to the J2000.0 ecliptic plane at epoch 2005 October 7. The uncertainties Trojan asteroids are small objects that share the semimajor axis at the 3 σ level in a, e, i are ± 0.1 AU, ± 0.01, and ± 0.1◦, respectively. of their host planet. They orbit the Sun with the same period as the planet and are said to be settled in the planet’s 1:1 mean mo- a (AU) ei(◦) Ω (◦) ω (◦) M (◦) tion resonance (MMR). -
Long-Term Evolution of the Neptune Trojan 2001 QR322
Mon. Not. R. Astron. Soc. 347, 833Ð836 (2004) Long-term evolution of the Neptune Trojan 2001 QR322 R. Brasser,1 S. Mikkola,1 T.-Y. Huang,2 P. Wiegert3 and K. Innanen4 1Tuorla Observatory, University of Turku, Piikkio,¬ Finland 2Deptartment of Astronomy, Nanjing University, Nanjing, China 3Astronomy Unit, Queen’s University, Kingston, ON, Canada 4Deptartment of Physics and Astronomy, York University, Toronto, ON, Canada Accepted 2003 October 1. Received 2003 September 9; in original form 2003 April 23 ABSTRACT We simulated more than a hundred possible orbits of the Neptune Trojan 2001 QR322 for the age of the Solar system. The orbits were generated randomly according to the probability density derived from the covariance matrix of the orbital elements. The test trajectories librate ◦ ◦ around Neptune’s L4 point, with amplitudes varying from 40 to 75 and libration periods varying from 8900 to 9300 yr. The ν 18 secular resonance plays an important role. There is a separatrix of the resonance so that the resonant angle switches irregularly between libration and circulation. The orbits are chaotic, with observed Lyapunov times from 1.7 to 20 Myr, approximately. The probability of escape to a non-Trojan orbit in our simulations was low, and only occurred for orbits starting near the low-probability edge of the orbital element distribution (largest values of initial semimajor axis and small eccentricity). This suggests that the Trojan may well be a primordial object. Key words: celestial mechanics Ð minor planets, asteroids Ð Solar system: general. in the element vector q were computed as 1 INTRODUCTION According to the Minor Planet Center, 1571 Trojan asteroids have 6 been discovered. -
Origin and Dynamical Evolution of Neptune Trojans – I: Formation and Planetary Migration
Origin and Dynamical Evolution of Neptune Trojans – I: Formation and Planetary Migration Patryk Sofia Lykawka,1* Jonathan Horner,2 Barrie W. Jones 2 and Tadashi Mukai1 1 Dept. of Earth and Planetary Sciences, Kobe University, 1-1 rokkodai-cho, nada-ku, Kobe 657- 8501, Japan 2 Dept. of Physics and Astronomy, The Open University, Walton Hall, Milton Keynes, MK7 6AA, UK ******* This paper was published in Monthly Notices of the Royal Astronomical Society 398, 1715-1729 (2009). [email protected] http://sites.google.com/site/patryksofialykawka/ ******* * Present address: International Center for Human Sciences (Planetary Sciences), Kinki University, 3-4-1 Kowakae, Higashiosaka-shi, Osaka-fu, 577-8502, Japan. E-mail address: [email protected] 1 of 25 ABSTRACT We present the results of detailed dynamical simulations of the effect of the migration of the four giant planets on both the transport of pre-formed Neptune Trojans, and the capture of new Trojans from a trans-Neptunian disk. The cloud of pre-formed Trojans consisted of thousands of massless particles placed on dynamically cold orbits around Neptune’s L4 and L5 Lagrange points, while the trans-Neptunian disk contained tens of thousands of such particles spread on dynamically cold orbits between the initial and final locations of Neptune. Through comparison of the results with previous work on the known Neptunian Trojans, we find that scenarios involving the slow migration of Neptune over a large distance (50 Myr to migrate from 18.1 AU to its current location, using an exponential-folding time of τ = 10 Myr) provide the best match to the properties of the known Trojans. -
Stability of Uranus and Neptune Trojans. the Case of 2001 QR322
A&A 410, 725–734 (2003) Astronomy DOI: 10.1051/0004-6361:20031275 & c ESO 2003 Astrophysics The MATROS project: Stability of Uranus and Neptune Trojans. The case of 2001 QR322 F. Marzari1, P. Tricarico1, and H. Scholl2 1 Dipartimento di Fisica, University of Padova, Via Marzolo 8, 35131 Padova, Italy 2 Observatoire de la Cˆote d’Azur, BP 4229, 06304 Nice Cedex 4, France Received 17 April 2003 / Accepted 8 August 2003 Abstract. We present in this paper an analysis of the long term stability of Trojan type orbits of both Uranus and Neptune. Employing the Frequency Map Analysis (hereinafter FMA) we measure the diffusion speed in the phase space for a large sample of Trojan orbits with short numerical integrations. High resolution diffusion maps are derived for different values of initial inclination. These maps outline where the most stable orbits can be found in the Trojan clouds of the two planets. The orbit of the newly discovered Neptune Trojan 2001 QR322 has been analysed in detail with the FMA method. In the phase space the body is located close to the border of a stable region for low inclination Neptune Trojans. Numerical integrations over 4.5 Gyr of clone orbits generated from the covariance matrix show that only 10% of the clones escape from the Trojan cloud. The proper frequencies of the Trojan motion computed with the FMA algorithm allow us to to derive a numerical secular theory. From this theory it is possible to locate in the phase space the main secular resonances that can perturb Trojan orbits of the two planets and lead to instability. -
Physical and Dynamical Study of Minor Bodies and Natural Satellites of the Solar System
PLANETARY SCIENCES: physical and dynamical study of minor bodies and natural satellites of the Solar System Monica Lazzarin The study of the minor bodies (asteroids, comets,trans-Neptunians, natural satellites etc.) is of particular relevance for understanding the formation process, the early phases and the subsequent dynamical, physical and chemical evolution of the Solar System. The lack of important modifications since their formation can give crucial clues on the status of the early solar nebula, and on the processes occurring some 4.5 billion years ago. Their observed diversity points to the existence of important thermal, dynamical and chemical gradients along the nebula that must also have affected the evolutionary history of the planets, and that requires a diversified approach for an overall vision. In particular, the following aspects that are subject of vast interest in the international community, constitute the scientific background of the present project (see references below of the research group and of other international groups on the subjects): A - Origin and nature of the Near Earth Asteroids/Objects (NEA/NEO). Their orbits come very close to that of the Earth, in several cases intersecting it and posing some impact threat. Their origin is not well known yet: they could be inactive/dormant comets, with a very pristine composition, but also more geologically processed asteroids. The NEA/NEOs population appears extremely heterogeneous in all their aspects: physical properties, shapes, albedos, surface compositions. A fraction (possibly large) of the total are binary systems; studies of their light curves demonstrate in some cases a complex rotational status, others show a long rotational period not easily explained in the framework of the current dynamical and collisional models. -
Formation and Dynamical Evolution of the Neptune Trojans the Influence of the Initial Solar System Architecture
Mon. Not. R. Astron. Soc. 404, 1272–1280 (2010) doi:10.1111/j.1365-2966.2010.16381.x Formation and dynamical evolution of the Neptune Trojans – the influence of the initial Solar system architecture P. S. Lykawka,1† J. Horner,2 B. W. Jones3 and T. Mukai4 1International Centre for Human Sciences (Planetary Sciences), Kinki University, 3-4-1 Kowakae, Higashiosaka, Osaka, 577-8502, Japan 2Department of Physics, Science Laboratories, University of Durham, South Road, Durham DH1 3LE 3Department of Physics and Astronomy, The Open University, Walton Hall, Milton Keynes MK7 6AA 4Kobe University, 1-1 rokkodai-cho, nada-ku, Kobe 657-8501, Japan Accepted 2010 January 19. Received 2010 January 19; in original form 2009 September 1 Downloaded from ABSTRACT Current models of Solar system formation suggest that the four giant planets accreted as a significantly more compact system than we observe today. In this work, we investigate the dynamical stability of pre-formed Neptune Trojans under the gravitational influence of the http://mnras.oxfordjournals.org/ four giant planets in compact planetary architectures, over 10 Myr. In our modelling, the initial orbital locations of Uranus and Neptune (aN) were varied to produce systems in which those planets moved on non-resonant orbits or in which they lay in their mutual 1:2, 2:3 and 3:4 mean-motion resonances (MMRs). In total, 420 simulations were carried out, examining 42 different architectures, with a total of 840 000 particles across all runs. In the non-resonant cases, the Trojans suffered only moderate levels of dynamical erosion, with the most compact systems (those with aN ≤ 18 au) losing around 50 per cent of their Trojans by the end of the integrations. -
Arxiv:1807.06783V1 [Astro-Ph.EP] 18 Jul 2018
Accepted for publication in the AJ. Preprint typeset using LATEX style emulateapj v. 01/23/15 THE CONTRIBUTION OF DWARF PLANETS TO THE ORIGIN OF LOW INCLINATION COMETS BY THE REPLENISHMENT OF MEAN MOTION RESONANCES IN DEBRIS DISKS. M. A. Munoz-Guti~ errez´ Institute of Astronomy and Astrophysics, Academia Sinica, National Taiwan University, Taipei, Taiwan A. Peimbert and B. Pichardo. Instituto de Astronom´ıa,Universidad Nacional Aut´onomade M´exico,Apdo. postal 70-264 Ciudad Universitaria, M´exico Accepted for publication in the AJ. ABSTRACT In this work we explore a new dynamical path for the delivery of low-inclination comets. In a con- figuration formed by an interior giant planet and an exterior massive debris disk, where the mass is accounted for by the 50 largest objects in the disk, the strongest mean motion resonances of the giant, located along the belt, are replenished with new material (test particles) due to the influence of the 50 massive objects. Once in resonance, slow chaotic diffusion stirs the orbital elements of the cometary nuclei enough to encounter the giant and to be scattered by it. When the disk is massive enough, both resonant and non-resonant particles are stirred quickly to encounter the giant and form an scattered disk component, greatly increasing the rate for the delivery of cometary material to the inner part of the system. This mechanism is applicable both to the solar system and extrasolar systems in general. Preliminary results, using a disk as massive as the classical Kuiper belt, indicate that the mechanism here proposed can account for about a tenth of the required injection rate to maintain the population of ecliptic comets in steady state. -
Appendix II. Publications
Appendix II. Publications Gemini Staff Publications Papers in Peer-Reviewed Journals: Trujillo, Chadwick A.[2]. Detection of a Trailing (L5) Neptune Trojan. Science, 329:1304-. September, 2010. Leggett, S. K.[2]. A Detailed Model Atmosphere Analysis of Cool White Dwarfs in the Sloan Digital Sky Survey. The Astrophysical Journal Supplement, 190:77-99. September, 2010. Schiavon, Ricardo P.[3]. Dissecting the Red Sequence. IV. The Role of Truncation in the Two- dimensional Family of Early-type Galaxy Star Formation Histories. The Astrophysical Journal, 721:278-296. September, 2010. Hayward, Thomas L.[7]; Hartung, Markus[12]. The Gemini NICI Planet-finding Campaign: Discovery of a Close Substellar Companion to the Young Debris Disk Star PZ Tel. The Astrophysical Journal Letters, 720:L82-L87. September, 2010. Leggett, S. K.[1]. Properties of the T8.5 Dwarf Wolf 940 B. The Astrophysical Journal, 720:252- 258. September, 2010. McDermid, R. M.[16]. Formation of slowly rotating early-type galaxies via major mergers: a resolution study. Monthly Notices of the Royal Astronomical Society, 406:2405-2420. August, 2010. Leggett, S. K.[4]. 47 new T dwarfs from the UKIDSS Large Area Survey. Monthly Notices of the Royal Astronomical Society, 406:1885-1906. August, 2010. Hartung, M.[3]. Observations of a stationary mid-latitude cloud system on Titan. Icarus, 208:868- 877. August, 2010. Geballe, T. R.[2]. High-resolution 3-μm spectra of Jupiter: Latitudinal spectral variations influenced by molecules, clouds, and haze. Icarus, 208:837-849. August, 2010. McDermid, Richard M.[3]; Miller, Bryan W.[5]. The Einstein Cross: Constraint on Dark Matter from Stellar Dynamics and Gravitational Lensing. -
Dynamics of the Kuiper Belt
To appear in Protostars and Planets IV Preprint (Jan 1999): http://www.lpi.usra.edu/science/renu/home.html DYNAMICS OF THE KUIPER BELT Renu Malhotra (1), Martin Duncan (2), and Harold Levison (3) (1) Lunar and Planetary Institute, (2) Queen’s University, (3) Southwest Research Institute Abstract. Our current knowledge of the dynamical objects (KBOs) have been discovered - a sufficiently large structure of the Kuiper Belt is reviewed here. Numeri- number that permits first-order estimates about the mass cal results on long term orbital evolution and dynamical and spatial distribution in the trans-Neptunian region. A mechanisms underlying the transport of objects out of the comparison of the observed orbital properties of these ob- Kuiper Belt are discussed. Scenarios about the origin of jects with theoretical studies provides tantalizing clues to the highly non-uniform orbital distribution of Kuiper Belt the formation and evolution of the outer Solar system. objects are described, as well as the constraints these pro- Other chapters in this book describe the observed physi- vide on the formation and long term dynamical evolution cal and orbital properties of KBOs (Jewitt and Luu) and of the outer Solar system. Possible mechanisms include their collisional evolution (Farinella et al); here we focus an early history of orbital migration of the outer planets, on the dynamical structure of the trans-Neptunian region a mass loss phase in the outer Solar system and scatter- and the dynamical evolution of bodies in it. The outline ing by large planetesimals. The origin and dynamics of of this chapter is as follows: in section II we review nu- the scattered component of the Kuiper Belt is discussed. -
Where Are the Uranus Trojans? R
Where are the Uranus Trojans? R. Dvorak, Á. Bazsó, L.-Y. Zhou To cite this version: R. Dvorak, Á. Bazsó, L.-Y. Zhou. Where are the Uranus Trojans?. Celestial Mechanics and Dynamical Astronomy, Springer Verlag, 2010, 107 (1-2), pp.51-62. 10.1007/s10569-010-9261-y. hal-00552504 HAL Id: hal-00552504 https://hal.archives-ouvertes.fr/hal-00552504 Submitted on 6 Jan 2011 HAL is a multi-disciplinary open access L’archive ouverte pluridisciplinaire HAL, est archive for the deposit and dissemination of sci- destinée au dépôt et à la diffusion de documents entific research documents, whether they are pub- scientifiques de niveau recherche, publiés ou non, lished or not. The documents may come from émanant des établissements d’enseignement et de teaching and research institutions in France or recherche français ou étrangers, des laboratoires abroad, or from public or private research centers. publics ou privés. Celest Mech Dyn Astr (2010) 107:51–62 DOI 10.1007/s10569-010-9261-y ORIGINAL ARTICLE Where are the Uranus Trojans? R. Dvorak · Á. Bazsó · L.-Y. Zhou Received: 11 November 2009 / Revised: 1 February 2010 / Accepted: 10 February 2010 / Published online: 11 March 2010 © Springer Science+Business Media B.V. 2010 Abstract The area of stable motion for fictitious Trojan asteroids around Uranus’ equila- teral equilibrium points is investigated with respect to the inclination of the asteroid’s orbit to determine the size of the regions and their shape. For this task we used the results of extensive numerical integrations of orbits for a grid of initial conditions around the points L4 and L5, and analyzed the stability of the individual orbits. -
Planetary Trojans – the Main Source of Short Period Comets?
Planetary Trojans – the main source of short period comets? J. Horner 1 & P. S. Lykawka 2 1 Department of Physics, Science Laboratories, University of Durham, South Road, Durham, UK, DH1 3LE 2 Astronomy Group, Faculty of Social and Natural Sciences, Kinki University, Shinkamikosaka 228-3, Higashiosaka-shi, Osaka, 577-0813, Japan ******* This paper has been accepted for publication in the International Journal of Astrobiology on 22 June 2010. [email protected] http://sites.google.com/site/patryksofialykawka/ ******* Abstract One of the key considerations when assessing the potential habitability of telluric worlds will be that of the impact regime experienced by the planet. In this work, we present a short review of our understanding of the impact regime experienced by the terrestrial planets within our own Solar system, describing the three populations of potentially hazardous objects which move on orbits that take them through the inner Solar system. Of these populations, the origins of two (the Near-Earth Asteroids and the Long-Period Comets) are well understood, with members originating in the Asteroid belt and Oort cloud, respectively. By contrast, the source of the third population, the Short- Period Comets, is still under debate. The proximate source of these objects is the Centaurs, a population of dynamically unstable objects that pass perihelion (closest approach to the Sun) between the orbits of Jupiter and Neptune. However, a variety of different origins have been suggested for the Centaur population. Here, we present evidence that at least a significant fraction of the Centaur population can be sourced from the planetary Trojan clouds, stable reservoirs of objects moving in 1:1 mean-motion resonance with the giant planets (primarily Jupiter and Neptune).