The Response of Detrital and Autotrophic Resources to Long-Term
Total Page:16
File Type:pdf, Size:1020Kb
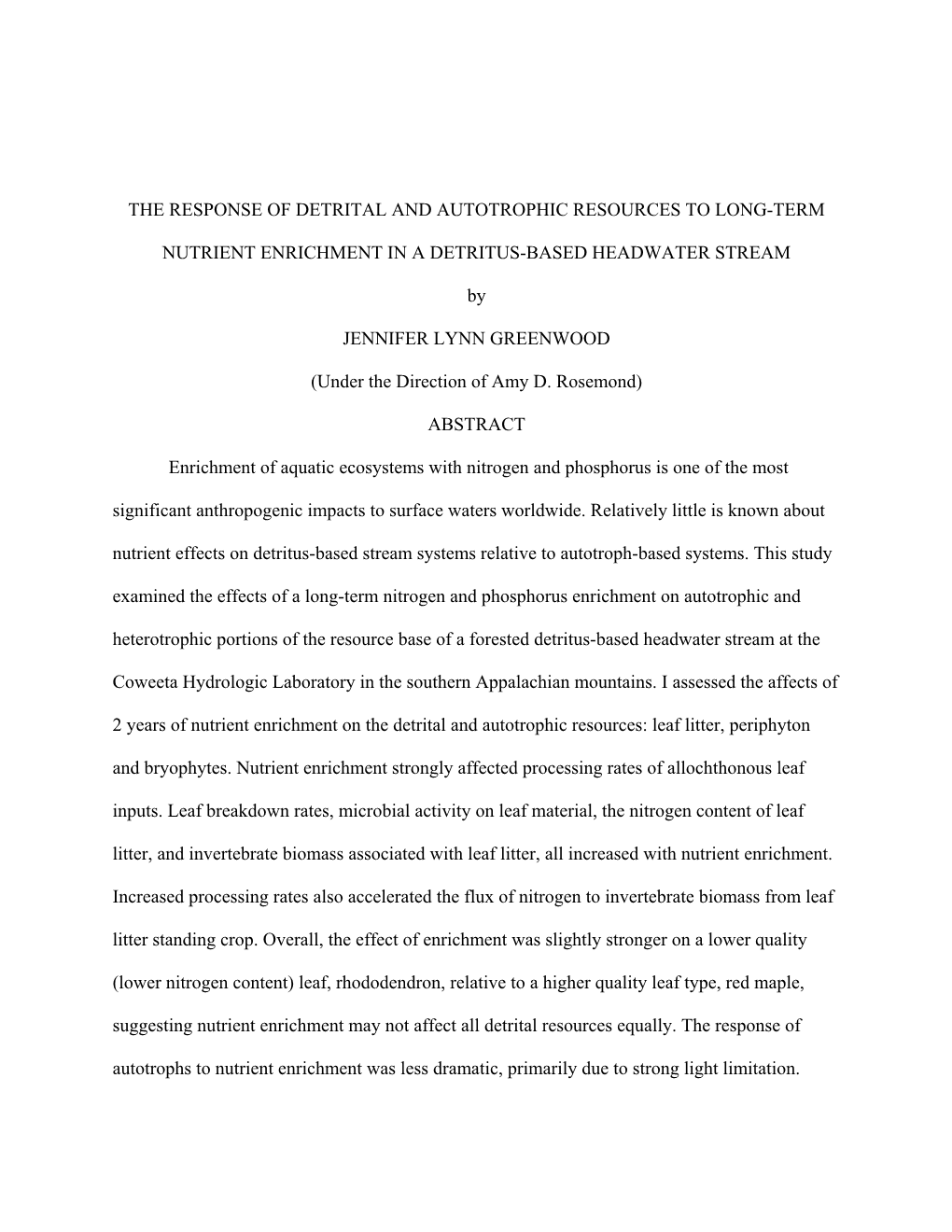
Load more
Recommended publications
-
Scientific and Engineering Advisory Panel Biographical Sketches
Scientific and Engineering Advisory Panel Biographical Sketches David Allan Professor Allan’s teaching interests are in the ecology of fresh waters, including their conservation, management, and restoration. He also helps lead interdisciplinary, team-taught courses and a University of Michigan academic minor on Global Environmental Change. He is the author of two widely used text books: Stream Ecology (2007, with M.M Castillo) and Streams: Their Ecology & Life (2001, with C.E. Cushing). Professor Allan’s current research investigates the influence of changing land use on river ecosystems, the factors affecting success of stream restoration, and the ecology of freshwater communities. In addition to both local and regional work centered in the Midwest, his research has taken place at sites in the Rocky Mountains, Southeast Alaska, Sweden, Israel, and Venezuela. Professor Allan’s conservation activities include working closely with local watershed councils, and he has served on the boards of American Rivers and the Michigan Chapter of The Nature Conservancy. He has frequently served as a consultant to the U.S. Environmental Protection Agency and other agencies on topics related to aquatic ecosystem health. Professor Allan is active professionally on many fronts and has held office in several professional societies, including the North American Benthological Society, the Society for Conservation Biology, and the Ecological Society of America. He is a Fellow of the American Association for the Advancement of Science and 2009 recipient of the Award of Excellence of the North American Benthological Society. David Allan received a Bachelor of Science degree (with honors) in Zoology from the University of British Columbia in 1966, and Master of Science and Doctoral degrees in Zoology from the University of Michigan in 1967 and 1971, respectively. -
John Stephen Kominoski
Last updated 24 January 2013 Department of Biological Sciences John Stephen Kominoski Southeast Environmental Research Center Florida International University 11200 SW 8th Street Miami, Florida 33199 USA [email protected] http://sites.google.com/site/jkominoski/ +1 305.348.7117 EDUCATION Ph.D. Ecology, Odum School of Ecology, University of Georgia, Athens, GA, USA 2008 Dissertation: Linking resource and consumer diversity to ecosystem function in a detritus-based watershed. Advisor: Catherine M. Pringle Graduate Teaching Portfolio Certificate, University of Georgia, Athens, GA, USA 2008 M.S. Biology, Loyola University Chicago, Chicago, IL, USA Thesis: Dissolved organic carbon from elevated CO2-altered leaf litter: impacts 2003 on stream periphyton growth and community structure. Advisor: Nancy C. Tuchman B.A. Biology, Augustana College, Rock Island, IL, USA 1999 ACADEMIC AND PROFESSIONAL APPOINTMENTS Assistant Professor, Department of Biological Sciences and Southeast Environmental 2013- Research Center, Florida International University, Miami, FL, USA Postdoctoral Research Associate, Odum School of Ecology, University of Georgia, Athens, 2010-2012 GA, USA Postdoctoral Research Fellow, Department of Forest Sciences, University of British Columbia, 2008-2010 Vancouver, BC, Canada Instructor of Record, Ecology, Interdisciplinary Field Program, University of Georgia, Athens, 2006- GA, USA Graduate Research Assistant, Odum School of Ecology, University of Georgia, Athens, GA 2003-2008 USA Graduate Research Assistant, Department of Biology, -
John Stephen Kominoski
Rosemond CV October 25, 2017 Amy Daum Rosemond rosemondlab.eology.uga.edu Google Scholar 140 East Green Street Athens, Georgia 30602706.542.3903 [email protected] EDUCATION 1993 Ph.D. Biology Vanderbilt University Advisors: Susan Brawley, Patrick J. Mulholland (Oak Ridge National Laboratory) 1987 M.A. Biology, University of North Carolina, Chapel Hill Advisor: Seth Reice 1982 B.S. Zoology, University of North Carolina, Chapel Hill APPOINTMENTS 2017-present Professor, Odum School of Ecology University of Georgia Athens, GA Interim Associate Dean of Administration 2011-2017 Associate Professor, Odum School of Ecology University of Georgia Athens, GA 2005-2011 Assistant Professor, Odum School of Ecology University of Georgia Athens, GA 1998-2005 Assistant Director, Institute of Ecology University of GeorgiaAthens, GA 1996-1998 Assistant Research Scientist, Institute of Ecology University of GeorgiaAthens, GA 1996-1997 Instructor, Institute of Ecology University of GeorgiaAthens, GA 1994-1997 National Science Foundation Postdoctoral Research Fellow, Institute of Ecology, University of Georgia AFFILIATIONS 2017-present UGA Institute for Resilient Infrastructure Systems 2015-present UGA River Basin Faculty 2014-present Center for Integrative Conservation Research Faculty Affiliate 2005-present UGA Water Faculty 2005-present Conservation Ecology Faculty AREAS OF RESEARCH Aquatic ecosystem ecology, global change effects on aquatic ecosystems, watershed urbanization, food webs, trophic ecology, nutrient effects in aquatic systems, ecological stoichiometry, detrital- based food webs, biodiversity and ecosystem function, headwater streams EDITORIAL BOARDS Ecology (Subject Matter Editor: 2011-present) Freshwater Biology (Editorial Board: 2001-2006) Rosemond CV PUBLICATIONS (75 total) Peer-Reviewed Journal Publications Graduate student authors are underlined, postdocsⱽ, undergraduate authors* 2018 Demi, L.M., J.P. -
Ecovoice 2014
THE UNIVERSITY OF GEORGIA ODUM SCHOOL OF ECOLOGY 2014 ANNUAL The Water Issue Notes from the Dean 2014 Annual Issue Dean John L. Gittleman Associate Dean for Academic Affairs Sonia Altizer JOHN GITTLEMAN Associate Dean for Administrative and External Affairs Laurie Fowler Editor and Writer Water, Water, Water Beth Gavrilles Contributing Writers nteresting scientific theorems often come via misconstrued lines or Lee Snelling phrases. One of the best, known as Aunt Jobiska’s theorem, comes from Katie Sheehan Ia poem by Edward Lear: “It’s a fact the whole world knows…” We know Graphic Designer the Earth is a watery place; water covers 71% of its surface. We know that Mary H. Andrews we must have water to live. And we know that continually and dramati- 706-542-2968 cally changing the flow, ecosystem structure, sustainable supply, health, or 706-542-4819 fax just about any other measurable aspect of water will lead to trouble. www.ecology.uga.edu Whenever something is so obvious, it’s open for full-throttle debate— especially when it involves an intrinsically important natural resource www.facebook.com/odumschool that’s unevenly distributed: of all the freshwater on Earth, 68 percent is in ice, 30 percent is in the ground, and, according to the U.N., over 780 mil- @UGAEcology lion people don’t have access to clean water. Dating back to the groundbreaking work of the Institute of Ecology, This publication is available in an over half of our faculty is dedicated to studying water-related issues. electronic format. Please contact Whether focused on species invasions along the coast, constructed the editor at [email protected] or wetlands for wastewater treatment, or the impacts of climate change and phone 706-542-7247. -
Craig A. Layman Ph.D., Ecology and Evolution PO Box 117 Maggie Valley, NC 28751 [email protected] 919 889 4262
Craig A. Layman Ph.D., Ecology and Evolution PO Box 117 Maggie Valley, NC 28751 [email protected] 919 889 4262 EDUCATION 2004 Ph.D. Texas A & M University, Ecology and Evolution Minor Concentration in Philosophy 1999 M.S. University of Virginia, Environmental Sciences 1996 B.A. University of Virginia, Triple Major: Biology, Economics, and Environmental Sciences PROFESSIONAL APPOINTMENTS 2017-2019 Professor, Chancellor’s Faculty Excellence Fellow, North Carolina State University 2013-2017 Associate Professor, Chancellor’s Faculty Excellence Fellow, North Carolina State University 2012-13 Associate Professor, Florida International University 2006-2012 Assistant Professor, Florida International University 2004-2006 Gaylord Donnelley Postdoctoral Fellow, Yale University 2003-2004 Fulbright Fellow, Venezuela EDITORSHIP 2019-Present Head Editor, Caribbean Naturalist (Eagle Hill Publications) 2019-Present Special Content Editor, Food Webs (Elsevier) 2019-Present Freelance Editor, Cactus Communications (India), Bioedit (UK), The Expert Editor (Australia), EditorBar (China) 2011-Present Editorial Board, Oecologia (Springer) 2016-2019 Editor-in-Chief, Food Webs (Elsevier) 2008-2019 Editorial Board, Caribbean Naturalist (Eagle Hill Publications) 2008-2011 Assistant Editor, PLoS One (Public Library of Science) 2 GRANTS AWARDED National Science Foundation, “Fish aggregations and biogeochemical hot spots across regional environmental gradients,” $657,849 (OCE 1405198, 2014-2019). National Geographic Society, “Exploring the role of biological factors in widespread mangrove die-off: A citizen science-based approach,” $29,800. National Fish and Wildlife Foundation, “New geographic and ecological connections between hawksbill nesting and foraging to bolster Caribbean conservation,” $25,000. National Science Foundation, “RAPID: An interdisciplinary approach to elucidating the causes of widespread mangrove die-off,” $75,187 (OCE 1405198, 2015-2016). -
Amy Daum Rosemond Rosemondlab.Ecology.Uga.Edu Google Scholar 140 East Green Street Athens, Georgia 30602706.542.3903 [email protected] Researcherid I-2688-2018
Rosemond CV March, 2020 Amy Daum Rosemond rosemondlab.ecology.uga.edu Google Scholar 140 East Green Street Athens, Georgia 30602706.542.3903 [email protected] ResearcherID I-2688-2018 EDUCATION 1993 Ph.D. Biology Vanderbilt University Advisors: Susan Brawley, Patrick J. Mulholland (Oak Ridge National Laboratory) 1987 M.A. Biology, University of North Carolina, Chapel Hill Advisor: Seth Reice 1982 B.S. Zoology, University of North Carolina, Chapel Hill APPOINTMENTS 2017-present Professor, Odum School of Ecology University of Georgia Athens, GA 2017 Interim Associate Dean of Administration and Research (March-December) 2011-2017 Associate Professor, Odum School of Ecology University of Georgia Athens, GA 2005-2011 Assistant Professor, Odum School of Ecology University of Georgia Athens, GA 1998-2005 Assistant Director, Institute of Ecology University of GeorgiaAthens, GA 1996-1998 Assistant Research Scientist, Institute of Ecology University of GeorgiaAthens, GA 1996-1997 Instructor, Institute of Ecology University of GeorgiaAthens, GA 1994-1997 National Science Foundation Postdoctoral Research Fellow, Institute of Ecology University of GeorgiaAthens, GA AFFILIATIONS 2017-present UGA Institute for Resilient Infrastructure Systems, Member and Advisory Board 2015-present UGA River Basin Center Faculty, Member and Advisory Board 2014-present Center for Integrative Conservation Research Faculty Affiliate 2005-present UGA Water Faculty 2005-present Conservation Ecology Faculty AREAS OF RESEARCH Aquatic ecosystem ecology, biogeochemistry, -
Futurity: the Next Five Years and Beyond Dean John Gittleman
2012 Vol. 3, No. 1 Futurity: The Next Five Years and Beyond Dean John Gittleman he future is here. What will be the Building on the strong foundation of the Richard B. Russell Award for Excellence in best ways to preserve our environ- Institute of Ecology, we have made remark- Undergraduate Teaching (Jeb Byers) and ments? When will we reach a tip- able strides in service, research, and teach- the Outstanding Advisor Award (Misha Tping point for global climate change? Will ing as well as creating a dynamic culture Boyd) from UGA—an extremely rare feat modern forms of communication (texts, that will allow us to grow, diversify, and for a single college; extraordinary for a Twitter, Skype…) fundamentally change adapt to rapidly changing ecological prob- unit of our size. the way we communicate, educate, think, lems. I want to mention a few accomplish- It’s now time for us to develop a new and solve problems? As a School of Ecol- ments of the past year that truly amaze: Strategic Plan that will set new intellectual ogy we must develop critical and effective Our students again topped the charts, and practical goals and challenges and ex- ways to be ready for such changes and to receiving two of UGA’s three Udall Schol- pand on our unique strengths, including: understand the impact they will have on arships (out of only 80 nationwide!); two • Intensely interdisciplinary work, an us and our planet. of UGA’s four Goldwater Scholarships; and “intermingling” of ideas and learning July 1, 2012 marked the five-year an- four of UGA’s ten NSF Graduate Research experiences. -
Taxonomic Identity Best Explains Variation in Body Nutrient Stoichiometry in a Diverse Marine Animal Community Jacob E
www.nature.com/scientificreports OPEN Taxonomic identity best explains variation in body nutrient stoichiometry in a diverse marine animal community Jacob E. Allgeier1*, Seth Wenger2 & Craig A. Layman3 Animal-mediated nutrient dynamics are critical processes in ecosystems. Previous research has found animal-mediated nutrient supply (excretion) to be highly predictable based on allometric scaling, but similar eforts to fnd universal predictive relationships for an organism’s body nutrient content have been inconclusive. We use a large dataset from a diverse tropical marine community to test three frameworks for predicting body nutrient content. We show that body nutrient content does not follow allometric scaling laws and that it is not well explained by trophic status. Instead, we fnd strong support for taxonomic identity (particularly at the family level) as a predictor of body nutrient content, indicating that evolutionary history plays a crucial role in determining an organism’s composition. We further fnd that nutrients are “stoichiometrically linked” (e.g., %C predicts %N), but that the direction of these relationships does not always conform to expectations, especially for invertebrates. Our fndings demonstrate that taxonomic identity, not trophic status or body size, is the best baseline from which to predict organismal body nutrient content. Animals are important drivers of nutrient dynamics in many ecosystems1–4. Nutrients frequently limit key ecosystem processes, such as primary production5, and animals can represent a major source of labile nutrients through their excretion, at times alleviating nutrient limitation 6–8. Because they are ofen the dominant pool of biomass (particularly in many aquatic systems), animals can also represent an important reservoir of nutrients stored in their tissue 9. -
2019 Annual Meeting Salt Palace Convention Center Floor Plans for the SFS 2019 Annual Meeting Contents Registration And
Garrett/Flickr.com 2019 Annual Meeting Salt Palace Convention Center Floor Plans for the SFS 2019 Annual Meeting Contents Registration and About SFS ..............................................................................................2–3 Check-In Information General Information ..........................................................................4–7 Registration and check in for the meeting will be available Schedule At-A-Glance ....................................................................... 8–10 all week in the North Foyer of the Salt Palace. Please check Plenary Speakers .................................................................................. 11 in upon your arrival at the meeting in order to receive Awards...............................................................................................12–13 your name badge and other important materials and information. Fellows Awards ................................................................................14–15 Exhibitors ..........................................................................................16–17 REGISTRATION HOURS Sunday, May 19—4:00pm to 10:00pm Sponsors.................................................................................................. 18 Monday, May 20—8:00am to 11:00pm Workshops .............................................................................................. 19 Tuesday, May 21—8:00am to 7:00pm Special Events ....................................................................................... -
Final Report: Stream Nutrient Responses
2013 LTER Synthesis Final Report: Stream nutrient responses FINAL REPORT “Stream ecosystem responses to nutrient enrichment at multiple trophic levels” LTER synthesis working group 2013 The goals of our working group were: (1) to initiate and coordinate the integration of data from multiple sites on stream ecosystem responses to nutrient amendment (2) to synthesize these data with a quantitative evaluation of functional responses at the microbial, primary producer, consumer and whole-stream levels (3) to promote interaction between LTER and the NEON experiment (Stream Experimental and Observatory Network, STREON) Participants included: Marcelo Ardon, East Carolina University (CWT) *co-PI Becky Bixby, University of New Mexico (SEV) *co-PI Jim Brock, Desert Research Institute Ayesha Burdett, New Mexico Museum of Natural History and Science Scott Cooper, University of Santa Barbara Walter Dodds, Kansas State University (KNZ) *co-PI Natalie Griffiths, Oak Ridge National Laboratory Nancy Grimm, Arizona State University (CAP) Tamara Harms, University of Alaska-Fairbanks (BNZ) Sherri Johnson, Oregon State University (HJA) *co-PI Jay Jones, University of Alaska-Fairbanks (BNZ) John Kominoski, Florida International University (FCE) Bill McDowell, University of New Hampshire (LUQ) Amy Rosemond, University of Georgia (CWT) *co-PI Jennifer Follstad-Shah, Utah State University Matt Trentman, Kansas State University (KNZ) Ryan Utz, National Ecological Observatory Network Dave Van Horn, University of New Mexico (MCM) Amy Ward, University of Alabama Lydia Zeglin, Oregon State University (HJA) *PI Our group met twice: Meeting 1: 2-day Workshop April 4-5, 2013, Boulder, CO Meeting 2: Lunch discussion May 21, 2013, Society for Freshwater Sciences (SFS) Annual Meeting, Jacksonville, FL; plus informal follow-up discussions during SFS Products include: 1. -
THE UNIVERSITY of GEORGIA ODUM SCHOOL of ECOLOGY 2015 ANNUAL Notes from the Dean
THE UNIVERSITY OF GEORGIA ODUM SCHOOL OF ECOLOGY 2015 ANNUAL Notes from the Dean 2015 Annual Issue Dean John L. Gittleman Associate Dean for Academic Affairs Sonia Altizer JOHN L. GITTLEMAN Associate Dean for Administrative and External Affairs Laurie Fowler Editor and Writer Beth Gavrilles Take a number Contributing Writers urrent rates of extinction are 1,000 times faster than historical Laura Early patterns; 16 percent of marine species are threatened; mainte- Lee Snelling Cnance of greenhouse gas emissions could result in 99 percent loss Graphic Designer of glaciers; 85 percent of endangered species are vulnerable to habitat loss; Mary H. Andrews 62 percent of human emerging diseases come from animals and plants. 706-542-2968 Numbers surround us and are, ecologically, often quite frightening! 706-542-4819 fax But not always. At Odum we’ve been seeing numbers that indicate www.ecology.uga.edu some very positive, optimistic trends. PROGRAM GROWTH www.facebook.com/odumschool We’re very excited about our new Ecology degree, a Bachelor of Arts. The AB has an emphasis on engagement, communication, learning skills and “experiential learning.” The new program builds on our core Ecology @UGAEcology courses that are shared with our existing BS degree, then adds problem solving and communication skills. The quotation marks around “expe- This publication is available in an riential learning” are to highlight that our new degree mirrors the UGA electronic format. Please contact the initiative to ensure that all undergraduates have some form of hands-on editor at [email protected] or phone learning. We’ve already taken the lead on this with 100 percent of our 706-542-7247. -
SFS Presentation Grid 2016
SFS Presentation Grid 2016 Time 302303 304305 306 307 308 309310 311312 313 314 315 Time/Location: Time/Location: Time/Location: Time/Location: Time/Location: Time/Location: Time/Location: Time/Location: Time/Location: Time/Location: [302303] – 10:30 [304305] – 10:30 [306] – 10:30 [307] – 10:30 [308] – 10:30 [309310] – 10:30 [311312] – 10:30 [313] – 10:30 [314] – 10:30 [315] – 10:30 Title: Title: Title: Title: Title: Title: Title: Title: Title: Title: CONTEMPORARY BEYOND LEVERAGING PRACTICAL LEVELS OF RESTORATION OF THE DOES COMPLIANCE FRESHWATER CADDISFLY WHAT PHYSIOLOGICAL EVALUATING WHEN AND HOW DYNAMIC THERMAL ADAPTATION RESOURCES: CITIZEN IDENTIFCATION FOR UPPER CLARK FORK WITH THE BRAZILIAN CONSERVATION IN BEHAVIORAL RESEARCH ON ION COMPENSATORY HYPORHEIC TEMPERATURE OF A PREDATOR SCIENCE OFFERS REAL LARVAE OF BAETIS RIVER, MT: FOREST CODE CENTRAL AMERICA RESPONSES TO DRYING TRANSPORT SUGGESTS MITIGATION UNDER MOSAICS INFLUENCE EXACERBATES SCIENCE AND REAL (EPHEMEROPTERA: OPPORTUNITIES FOR MITIGATE THE IMPACTS AND THE CARIBBEAN CUES IN TEMPORARY ABOUT THE POTENTIAL THE CLEAN WATER ACT: CHANNEL TEMPERATURE Sunday ECOLOGICAL DATA TO ADDRESS AND BAETIDAE) IN NORTH SYNERGY OF SUGARCANE PONDS: IMPLICATIONS TOXICITY OF SULFATE THE STATE OF THE REGIMES 10:30 CONSEQUENCES OF SOLVE REAL PROBLEMS AMERICA AGRICULTURE AND ITS Authors: FOR EFFECTS OF SCIENCE CLIMATE WARMING Authors: LEGACY ON INSTREAM Alonso Ramirez CLIMATE CHANGE Authors: Authors: Authors: Authors: H. Maurice Valett, Marc NUTRIENT Michael Griffith Authors: Katie Fogg,