High-Pressure Studies of Analogs with Applications to Materials Science
Total Page:16
File Type:pdf, Size:1020Kb
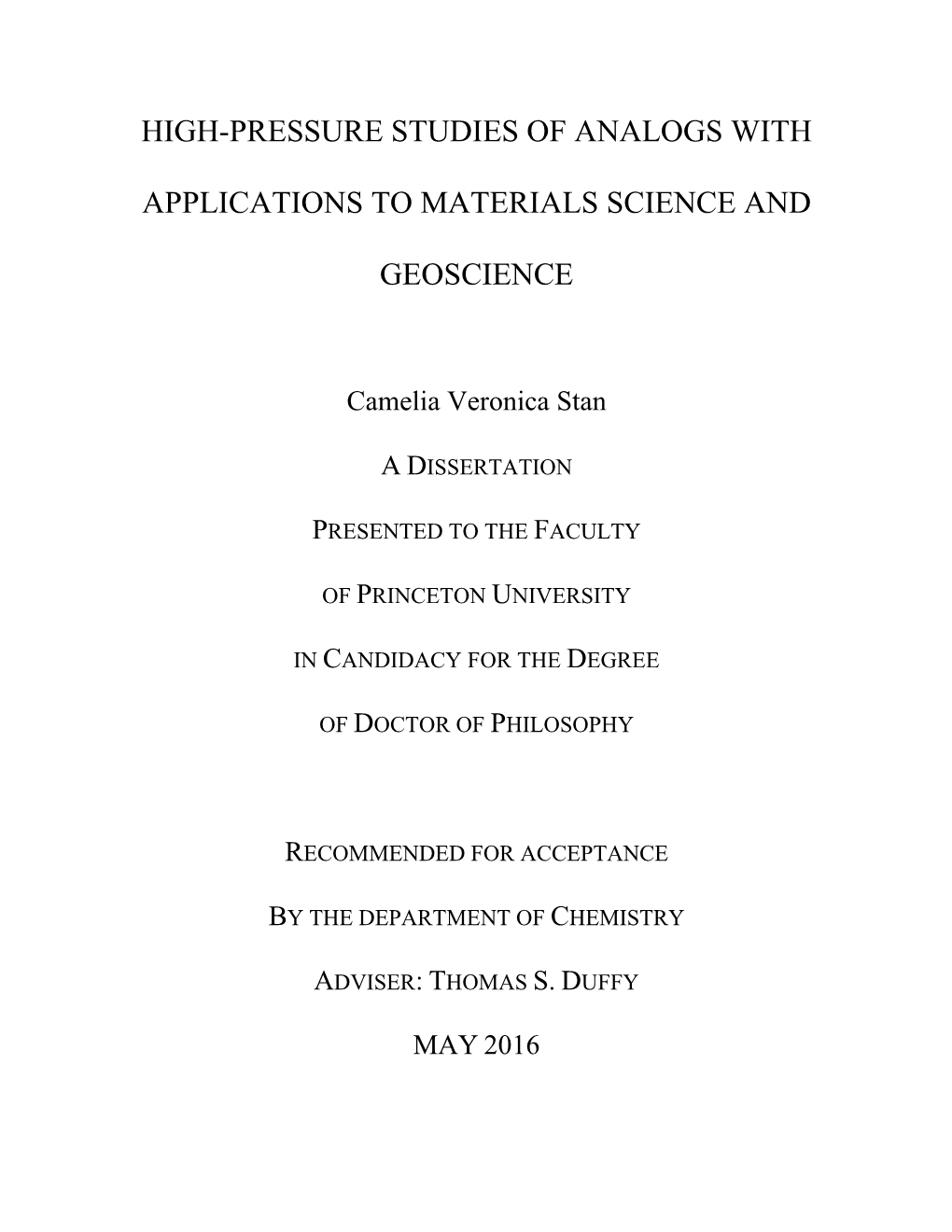
Load more
Recommended publications
-
A Review of the Melting Curves of Transition Metals at High Pressures Using Static Compression Techniques
crystals Review A Review of the Melting Curves of Transition Metals at High Pressures Using Static Compression Techniques Paraskevas Parisiades CNRS UMR 7590, Muséum National d’Histoire Naturelle, Institut de Minéralogie de Physique des Matériaux et de Cosmochimie, Sorbonne Université, 4 Place Jussieu, F-75005 Paris, France; [email protected] Abstract: The accurate determination of melting curves for transition metals is an intense topic within high pressure research, both because of the technical challenges included as well as the controversial data obtained from various experiments. This review presents the main static techniques that are used for melting studies, with a strong focus on the diamond anvil cell; it also explores the state of the art of melting detection methods and analyzes the major reasons for discrepancies in the determination of the melting curves of transition metals. The physics of the melting transition is also discussed. Keywords: melting curves; laser-heated diamond anvil cell; extreme conditions; synchrotron radia- tion; transition metals; phase transitions 1. Introduction Transition metals are defined as those elements that have a partially filled d-electron Citation: Parisiades, P. A Review of sub-shell. The strong metallic bonding due to the delocalization of d-orbitals is responsible the Melting Curves of Transition for a series of very interesting properties, such as high yield strength, corrosion and Metals at High Pressures Using Static wear resistance, good ductility, easy alloy and metallic glass formation, paramagnetism, Compression Techniques. Crystals 2021, 11, 416. https://doi.org// and high melting points and molar enthalpies of fusion, leading to a plethora of industrial 10.3390cryst11040416 applications [1–6]. -
Experimental Mineralogy and Mineral Physics - Ronald Miletich
GEOLOGY – Vol. III - Experimental Mineralogy and Mineral Physics - Ronald Miletich EXPERIMENTAL MINERALOGY AND MINERAL PHYSICS Ronald Miletich ETH Zürich, Switzerland Keywords: experimental mineralogy, mineral transformations, polymorphism, order– disorder, exsolution, high-pressure autoclaves, large-volume presses, diamond-anvil cell, static and dynamic pressure generation, in situ measurements, mineral physics, structure-property relations, physical anisotropy, tensor formalism, transport properties, electric and thermal conductivity, atomic diffusion, elasticity, dielectric polarization, refractive index, birefringence, optical activity, thermal expansion, pyroelectricity, piezoelectricity Contents 1. Experimental Mineralogy 1.1. Indirect Observation through the Experiment 1.2. Mineral Transformations 1.2.1. Polymorphism 1.2.2. Order–Disorder Transformations 1.2.3. Mineral Reactions 1.3. Experimental High-Pressure–High-Temperature Techniques 1.3.1. Large-Volume Autoclaves 1.3.2. Piston-Cylinder Technique 1.3.3. Multi-Anvil Press 1.3.4. Diamond-Anvil Cell 1.3.5. Shock-Wave Experiments 1.4. In Situ Measurements 1.4.1. Diffraction Techniques 1.4.2. Spectroscopy and Microscopy 2. Mineral Physics 2.1. Physical Properties of Crystals 2.1.1. Isotropy–Anisotropy 2.1.2. Mathematical Description: Tensors 2.2. Transport Properties 2.2.1. ThermalUNESCO Conductivity – EOLSS 2.2.2. Atomic Diffusion 2.2.3. Electrical Conductivity 2.3. Elastic propertiesSAMPLE CHAPTERS 2.3.1. Elastic Moduli 2.3.2. Methods for Determination of Elastic Moduli 2.4. Optical Properties 2.4.1. Refractive Index and Optical Indicatrix 2.4.2. Polarization and Birefringence 2.4.3. Optical Activity 2.5. Thermomechanical and Electromechanical Properties 2.5.1. Thermal Expansion 2.5.2. Pyroelectricity ©Encyclopedia of Life Support Systems (EOLSS) GEOLOGY – Vol. -
The 5-Th Element a New High Pressure High Temperature Allotrope
The 5-th element A new high pressure high temperature allotrope Von der Fakultät für Chemie und Geowissenschaften Der Universität Bayreuth zur Erlangung der Würde eines Doctors der Naturwissenschaften -Dr. rer. nat.- Dissertation vorgelegt von Evgeniya Zarechnaya aus Moskau (Russland) Bayreuth, April 2010 TABLE OF CONTENTS SUMMARY................................................................................................................................................... 1 ZUSAMMENFASSUNG.............................................................................................................................. 3 1 INTRODUCTION....................................................................................................................... 6 1.1 History of the discovery of boron................................................................................................... 6 1.2 Basic chemistry and crystal-chemistry of boron........................................................................... 7 1.3 Boron in natural systems ................................................................................................................ 9 1.4 Physical properties of boron and its application ........................................................................ 12 1.5 Boron at high pressure.................................................................................................................. 15 1.6 Experimental techniques and sample characterization ............................................................ -
A Large Volume Cubic Press with a Pressure-Generating Capability up to About 10 Gpa
High Pressure Research Vol. 32, No. 2, June 2012, 239–254 A large volume cubic press with a pressure-generating capability up to about 10 GPa Xi Liua,b*, Jinlan Chena,b,c, Junjie Tanga,b, Qiang Hea,b, Sicheng Lia,b,c, Fang Pengd, Duanwei Hed, Lifei Zhanga,b and Yingwei Feia,b,e aKey Laboratory of Orogenic Belts and Crustal Evolution, Ministry of Education of China, Beijing, People’s Republic of China; bSchool of Earth and Space Sciences, Peking University, Beijing 100871, Beijing, People’s Republic of China; cHenan Sifang Diamond Co., Ltd, Zhengzhou 450016, People’s Republic of China; dInstitute of Atomic and Molecular Physics, Sichuan University, Chengdu 610065, People’s Republic of China; eGeophysical Laboratory, Carnegie Institution of Washington, 5251 Broad Branch Road, NW, Washington, DC 20015, USA (Received 19 August 2011; final version received 11 January 2012) A massive cubic press, with a maximum load of 1400 tons on every WC anvil, has been installed at the High Pressure Laboratory of Peking University. High-P experiments have been conducted to examine the per- formance of the conventional experimental setup and some newly developed assemblies adopting the anvil-preformed gasket system. The experimental results suggest that (1) the conventional experimental setup (assembly BJC2-0) can reach pressures up to about 6 GPa with a large cell volume of 34.33 cm3; (2) the anvil-preformed gasket system, despite decreasing the P-generating efficiency, extends the P-generating capability up to about 8 GPa at the expense of reducing the cell volume down to 8.62 cm3 (assembly BJC2- 6); (3) due to the large cell volume, it is possible to make further modifications to extend the pressure range, as readily demonstrated, to about 10 GPa (assembly BJC5-7); (4) the effect of high temperature on the pressure generation of the press is not significant. -
© 2014 Jin Zhang
© 2014 Jin Zhang NEW HIGH PRESSURE PHASE TRANSITION OF NATURAL ORTHOENSTATITE AND SOUND VELOCITY MEASUREMENTS AT SIMULTANEOUS HIGH PRESSURES AND TEMPERATURES BY LASER HEATING BY JIN ZHANG DISSERTATION Submitted in partial fulfillment of the requirements for the degree of Doctor of Philosophy in Geology in the Graduate College of the University of Illinois at Urbana-Champaign, 2014 Urbana, Illinois Doctoral Committee: Professor Jay D. Bass, Chair and Director of Research Professor Xiaodong Song Assistant Professor Lijun Liu Associate Professor Przemyslaw Dera , University of Hawaii Abstract Studies of phase transformations and sound velocities of candidate mantle minerals under high-pressure high-temperature conditions are essential for understanding the mineralogical composition and physical processes of Earth’s interior. Phase transitions in candidate deep earth minerals under high-pressure high-temperature conditions is one of the main causes of the seismically observed discontinuities that define the boundaries between major layers of the Earth. The changes of sound velocities, including the directional dependencies of sound velocities, across phase transitions are still not well constrained. This is largely due to the lack of sound velocity measurements at sufficiently high pressure-temperature conditions and/or using only polycrystalline instead of single-crystal samples. To address the issues mentioned above, my dissertation involves two main topics Firstly, I discovered a new high-pressure Pbca -P2 1/c phase transition of natural orthoenstatite (Mg,Fe)SiO 3 using synchrotron single-crystal X-ray diffraction. This discovery contradicts with the widely accepted phase diagram of (Mg,Fe)SiO 3. I performed additional in situ Raman spectroscopy experiments addressing both compositional and high-temperature effects of this phase transition, providing solid evidences for the stability of orthoenstatite ( Pbca ) under average upper-most mantle conditions, and a potential stability/metastablity field for the new high-pressure P2 1/c phase. -
Anvil Cell Gasket Design for High Pressure Nuclear Magnetic Resonance Experiments Beyond 30 Gpa Thomas Meier, and Jürgen Haase
Anvil cell gasket design for high pressure nuclear magnetic resonance experiments beyond 30 GPa Thomas Meier, and Jürgen Haase Citation: Review of Scientific Instruments 86, 123906 (2015); doi: 10.1063/1.4939057 View online: https://doi.org/10.1063/1.4939057 View Table of Contents: http://aip.scitation.org/toc/rsi/86/12 Published by the American Institute of Physics Articles you may be interested in Moissanite anvil cell design for giga-pascal nuclear magnetic resonance Review of Scientific Instruments 85, 043903 (2014); 10.1063/1.4870798 High sensitivity nuclear magnetic resonance probe for anvil cell pressure experiments Review of Scientific Instruments 80, 073905 (2009); 10.1063/1.3183504 Nuclear magnetic resonance in a diamond anvil cell at very high pressures Review of Scientific Instruments 69, 479 (1998); 10.1063/1.1148686 Magnetic flux amplification by Lenz lenses Review of Scientific Instruments 84, 085120 (2013); 10.1063/1.4819234 A cubic boron nitride gasket for diamond-anvil experiments Review of Scientific Instruments 79, 053903 (2008); 10.1063/1.2917409 Theory of the gasket in diamond anvil high-pressure cells Review of Scientific Instruments 60, 3789 (1989); 10.1063/1.1140442 REVIEW OF SCIENTIFIC INSTRUMENTS 86, 123906 (2015) Anvil cell gasket design for high pressure nuclear magnetic resonance experiments beyond 30 GPa Thomas Meier and Jürgen Haase Faculty of Physics and Earth Sciences, University of Leipzig, Linnéstrasse 5, Leipzig 04103, Germany (Received 6 November 2015; accepted 15 December 2015; published online 31 December 2015) Nuclear magnetic resonance (NMR) experiments are reported at up to 30.5 GPa of pressure using radiofrequency (RF) micro-coils with anvil cell designs. -
NSF Renewal Proposal Appendix A
APPENDIX A: Table of Contents COMPRES Facilities 1. ALS 12.2.2 Beamline DAC (PI: Quentin Williams, UC Santa Cruz) 2. APS Beamline 13BM-C Partnership for Extreme Xtallography (PX^2) DAC (PI: Przemek Dera, Univ. Hawaii) 3. APS Gas Loading for DAC (PI: Mark Rivers, Univ. Chicago) 4. APS Nuclear Resonant and Inelastic X-Ray Scattering at Sector 3-ID DAC, Mössbauer (PI: Jay Bass, UIUC) 5. Multi-anvil Project (PI: Kurt Leinenweber, ASU) 6. NSLS-II Frontier Infrared Spectroscopy (FIS) Facility for Studies under Extreme Conditions DAC (PIs: Russell Hemley, Zhenxian Liu, GWU) 7. NSLS-II XPD & APS 6BM-B Beamlines MAP (PI: Don Weidner, Stony Brook) New Facilities proposed for COMPRES IV 1. CETUS at NASA Johnson Space Center (PI: Lisa Danielson, Jacobs Technology) 2. Large Multi-Anvil Press (LMAP) at Arizona State (PI: Kurt Leinenweber, ASU) New EOID projects proposed for COMPRES IV 1. A Career Path for African-American Students from HBCUs to National Laboratories (PI: Bob Liebermann, Stony Brook) 2. Infrastructural Development for Deep-Earth Large-Volume Experimentation “DELVE” (PI: Yanbin Wang, U. Chicago) 3. Development of an Electrical Cell in the Multi-Anvil to Study Planetary Deep Interiors (PI: Anne Pommier, UC San Diego) 1 COMPRES Facilities 1. ALS 12.2.2 Beamline DAC (PI: Quentin Williams, UC Santa Cruz) Over the next five years, we plan to continue to augment the portfolio of the Earth Sciences high- pressure effort at the Lawrence Berkeley National Labs Advanced Light Source, while continuing to maintain the standard capabilities that have served our user community well. -
Experimental Techniques Tools I: Diamond Anvil Cells
Experimental techniques Tools I: Diamond Anvil Cells Experimental Petrology (0 – 40 GPa, 25-3000°C) Experimental Mineral Physics (0-350 GPa, 25-7000°C) sintered diamond cube multi anvil Diamond Anvil Cells (DAC) 0 – 350 GPa, 7000°C gas pressures Tools I: Diamond Anvil Cells Tools I: Diamond Anvil Cells 1 Tools I: Diamond Anvil Cell • extreme conditions • in-situ observations (spectroscopy, X-ray diffraction etc etc) • extreme temperature gradients • open chemical system • micron-size samples • good for simple systems and mineral physics questions • not so good for petrology and phase diagrams Future: • since a few years chemical analyses of run products with FIP - analytical TEM Tools II: Gas pressures Tools III: Piston Cylinder Solid Media Presses Typically 5 kbar, 600 oC Very slow quench Two types: externally heated (pressure vessel at high T) internally heated (furnace inside the water cooled pressure vessel) 320t Piston Cylinder 0.5 - 4 GPa, 2000°C typically 4-5 kbar, 1300 oC, a few (one ?) to 12 kbar, 1200 oC 2 The Centrifuging Piston Cylinder Tools III: piston cylinders 25 cm, 38 kg - typical magmatic pressures: 2-18 kbar ( 5 - 55 km depth ) - standard apparatus: piston cylinder (with standard size of 80-200 cm height and 200-500 kg weight) Tools 3: piston cylinders Endloaded = two hydraulic cylinders, one for piston, one to pre-constrain ("loading") the pressure vessel axially Single stage = one hydraulic cylinder to drive piston conical steel ring pressed into matrix provides lateral stress/pre-loading piston cylinders are relatively low-tech, reliable (>95% technically successful experiments) not so expensive (200 SFr/experiment) - the work horses of experimental petrology 3 Belt apparatus - concave shaped bore/cell - max. -
Pressure and High-Temperature Studies of the Fe-C System
CARBON IN DEEP EARTH FROM HIGH- PRESSURE AND HIGH-TEMPERATURE STUDIES OF THE FE-C SYSTEM A DISSERTATION SUBMITTED TO THE GRADUATE DIVISION OF THE UNIVERSITY OF HAWAI‘I AT MĀNOA IN PARTIAL FULFILLMENT OF THE REQUIREMENTS FOR THE DEGREE OF DOCTOR OF PHILOSOPHY IN GEOLOGY AND GEOPHYSICS May 2019 By Xiaojing Lai Dissertation Committee: Bin Chen, Chairperson Przemyslaw Dera Julia E. Hammer Jasper Konter Michael J. Mottl Copyright © 2019, Xiaojing Lai ii This dissertation is dedicated to my loving and supporting husband, Feng Zhu and parents, Xiaoli Tang, Xulong Lai iii Acknowledgments My thanks to my supervisor Dr. Bin Chen are endless. He supported and encouraged me through the past five years of my Ph.D. studies. He taught me that doing experiment is a painstaking task and every small step matters. He showed me how to be an independent researcher and how to write and publish my research by providing valuable insights and feedback. I am also grateful to my committee members Dr. Przemyslaw Dera, Julia Hammer, Jasper Konter and Michael Mottl. Przemek also taught me a lot and helped me with the collection and analysis of the single-crystal X-ray diffraction data. Julia was the teacher of my mineralogy and theoretical petrology classes, I always enjoyed and learned a lot from her class. Jasper and Mike are also very helpful, their insights, comments and the questions raised in the every-semester dissertation committee meetings improve my dissertation a lot. I am also thankful for my comprehensive committee members: Drs. Eric Hellebrand, Jeff Taylor, and Shiv Sharma; teachers: Drs. -
Burlington House Fire Safety Information
Deep Earth Processes 2014 Contents Page 2 Sponsors acknowledgement Page 3 Oral Presentation Programme Page 8 Oral Presentation Abstracts Page 74 Posters Page 77 Poster Presentation Abstracts Page 102 Burlington House Fire Safety Information Page 103 Ground Floor Plan of The Geological Society Burlington House Page 104 2014 Geological Society Conferences 15-16 September 2014 Page 1 #deepearth14 Deep Earth Processes 2014 We gratefully acknowledge the support of the sponsors for making this meeting possible. 15-16 September 2014 Page 2 #deepearth14 Deep Earth Processes 2014 Oral Presentation Programme Monday 15 Sept 2014 08.30 Registration & tea & coffee (Main foyer & Lower Library) 09.05 Welcome Session 1: EARTH’S CORE (Chair: Dr Sally Gibson. Sponsor: British Geophysical Association) 09.10 Three potential solutions to the Core heat paradox Keynote: John Hernlund (Tokyo Institute of Technology, Japan) 09.40 Contstraints on the timing of late accretion from highly siderophile elements and W isotopes in the early rock record Chris Dale (University of Durham, UK) 10.00 Fe-Silicide-bearing Ureilite Parent Body: an analogue for the early building blocks of the Earth? Hilary Downes (University College London_Birkbeck, UK) 10.20 Making the Moon from the Earth – an internally consistent isotopic and chemical model Jon Wade (University of Oxford, UK) 10.40 Tea, coffee, refreshments & posters (Lower Library) 15-16 September 2014 Page 3 #deepearth14 Deep Earth Processes 2014 Session 2: STRUCTURE & COMPOSITION OF EARTH’S CORE (Chair: Prof Simon Redfern. Sponsor: Cambridge University Press) 11.10 Seismic structure of the Earth’s inner core and its dynamical implications Invited: Arwen Deuss (University of Cambridge, UK) 11.40 The melting curve of Ni to 1 Mbar Oliver Lord (University of Bristol, UK) 12.00 Development of an early density stratification in the Earth’s Core David Rubie (University of Bayreuth, Germany) 12.20 Discussion 12.35 Sandwich lunch (provided) & posters (Lower Library) Session 3: LOWERMOST MANTLE (Chair: Prof Simon Redfern. -
Poirier J.-P. Introduction to the Physics of the Earth`S Interior (2Ed
Introduction to the Physics of the Earth’s Interior describes the structure, composition and temperature of the deep Earth in one comprehensive volume. The book begins with a succinct review of the fundamentals of continu- um mechanics and thermodynamics of solids, and presents the theory of lattice vibration in solids. The author then introduces the various equations of state, moving on to a discussion of melting laws and transport properties. The book closes with a discussion of current seismological, thermal and compositional models of the Earth. No special knowledge of geophysics or mineral physics is required, but a background in elementary physics is helpful. The new edition of this successful textbook has been enlarged and fully updated, taking into account the considerable experimental and theoretical progress recently made in understanding the physics of deep- Earth materials and the inner structure of the Earth. Like the first edition, this will be a useful textbook for graduate and advanced undergraduate students in geophysics and mineralogy. It will also be of great value to researchers in Earth sciences, physics and materials sciences. Jean-Paul Poirier is Professor of Geophysics at the Institut de Physique du Globe de Paris, and a corresponding member of the Acade´mie des Sciences. He is the author of over one-hundred-and-thirty articles and six books on geophysics and mineral physics, including Creep of Crystals (Cambridge University Press, 1985) and Crystalline Plasticity and Solid-state flow of Metamorphic Rocks with A. Nicolas (Wiley, 1976). MMMM INTRODUCTION TO THE PHYSICS OF THE EARTH’S INTERIOR SECOND EDITION JEAN-PAULPOIRIER Institut de Physique du Globe de Paris The Pitt Building, Trumpington Street, Cambridge, United Kingdom The Edinburgh Building, Cambridge CB2 2RU, UK http://www.cup.cam.ac.uk 40 West 20th Street, New York, 10011-4211, USA http://www.cup.org 10 Stamford Road, Oakleigh, Melbourne 3166, Australia Ruiz de Alarco´n 13, 28014 Madrid, Spain © Jean-Paul Poirier 2000 This book is in copyright. -
High Pressure and High Temperature Behavior of Tialn
Linköping Studies in Science and Technology Licentiate Thesis No. 1540 High pressure and high temperature behavior of TiAlN Niklas Norrby LIU-TEK-LIC-2012:25 NANOSTRUCTURED MATERIALS DEPARTMENT OF PHYSICS, CHEMISTRY AND BIOLOGY (IFM) LINKÖPING UNIVERSITY SWEDEN Niklas Norrby ISBN: 978-91-7519-863-7 ISSN: 0280-7971 Printed by LiU-Tryck, Linköping, Sweden, 2012 Abstract This licentiate thesis mainly reports about the behavior of arc evaporated TiAlN at high pressures and high temperatures. The extreme conditions have been obtained in metal cutting, multi anvil presses or diamond anvil cells. Several characterization techniques have been used, including x-ray diffraction and transmission electron microscopy. Results obtained during metal cutting show that the coatings are subjected to a peak normal stress in the GPa region and temperatures around 900 °C. The samples after metal cutting are shown to have a stronger tendency towards the favorable spinodal decomposition compared to heat treatments at comparable temperatures. We have also shown an increased anisotropy of the spinodally decomposed domains which scales with Al composition and results in different microstructure evolutions. Furthermore, multi anvil press and diamond anvil cell at even higher pressures and temperatures (up to 23 GPa and 2200 °C) also show that the unwanted transformation of cubic AlN into hexagonal AlN is suppressed with an increased pressure and/or temperature. i ii Preface This is a summary of my work between April 2010 and April 2012. During these years, the main focus of my work has been to study the behavior of arc evaporated TiAlN at high pressures and high temperatures with the key results presented in the appended papers.