Direct Allylic C–H Alkylation of Enol Silyl Ethers Enabled by Photoredox–
Total Page:16
File Type:pdf, Size:1020Kb
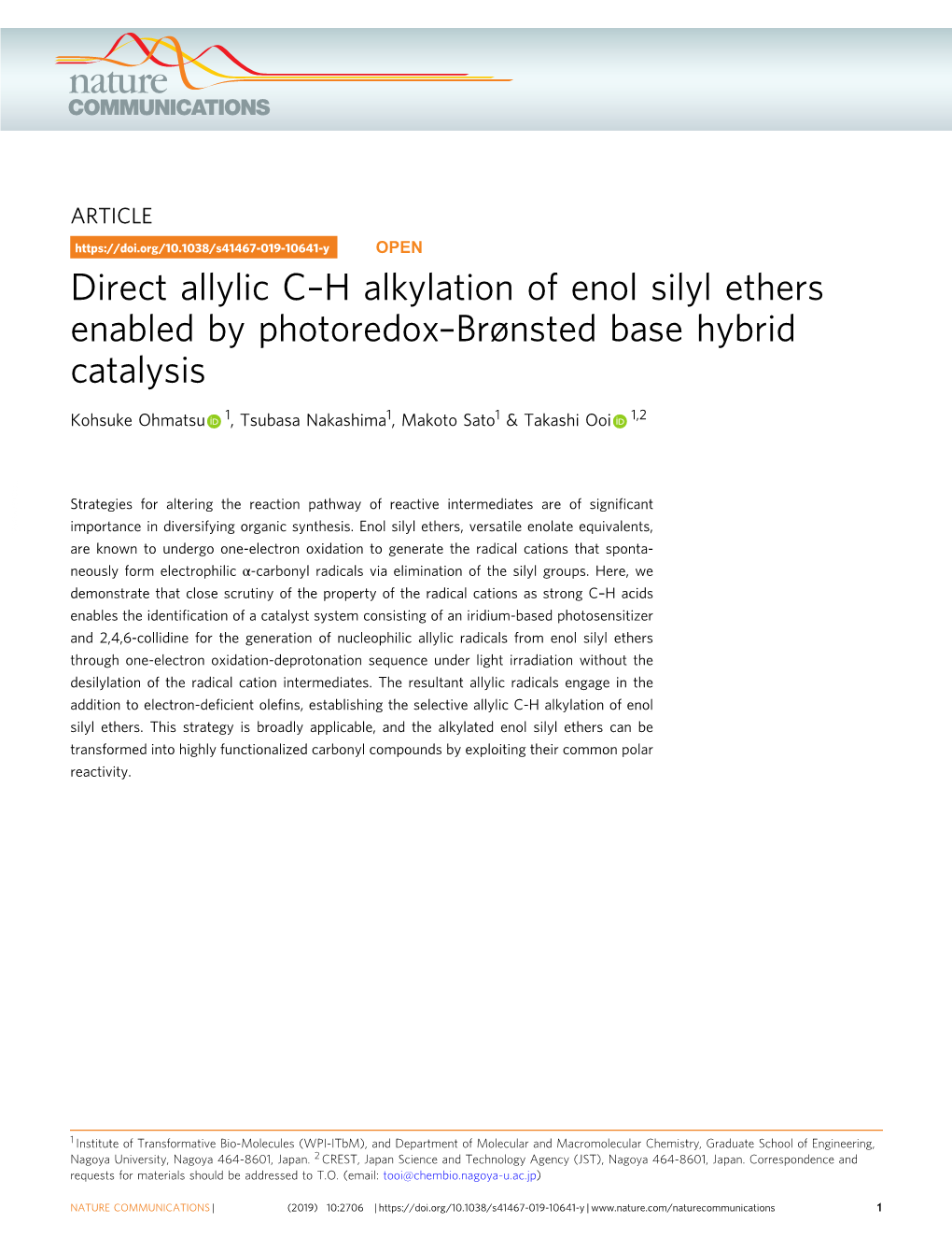
Load more
Recommended publications
-
Activation of Silicon Bonds by Fluoride Ion in the Organic Synthesis in the New Millennium: a Review
Activation of Silicon Bonds by Fluoride Ion in the Organic Synthesis in the New Millennium: A Review Edgars Abele Latvian Institute of Organic Synthesis, 21 Aizkraukles Street, Riga LV-1006, Latvia E-mail: [email protected] ABSTRACT Recent advances in the fluoride ion mediated reactions of Si-Η, Si-C, Si-O, Si-N, Si-P bonds containing silanes are described. Application of silicon bonds activation by fluoride ion in the syntheses of different types of organic compounds is discussed. A new mechanism, based on quantum chemical calculations, is presented. The literature data published from January 2001 to December 2004 are included in this review. CONTENTS Page 1. INTRODUCTION 45 2. HYDROSILANES 46 3. Si-C BOND 49 3.1. Vinyl and Allyl Silanes 49 3.2. Aryl Silanes 52 3.3. Subsituted Alkylsilanes 54 3.4. Fluoroalkyl Silanes 56 3.5. Other Silanes Containing Si-C Bond 58 4. Si-N BOND 58 5. Si-O BOND 60 6. Si-P BOND 66 7. CONCLUSIONS 66 8. REFERENCES 67 1. INTRODUCTION Reactions of organosilicon compounds catalyzed by nucleophiles have been under extensive study for more than twenty-five years. In this field two excellent reviews were published 11,21. Recently a monograph dedicated to hypervalent organosilicon compounds was also published /3/. There are also two reviews on 45 Vol. 28, No. 2, 2005 Activation of Silicon Bonds by Fluoride Ion in the Organic Synthesis in the New Millenium: A Review fluoride mediated reactions of fluorinated silanes /4/. Two recent reviews are dedicated to fluoride ion activation of silicon bonds in the presence of transition metal catalysts 151. -
Photoredox Catalysis Photoredox Catalysis: a Mild, Operationally Simple Approach to the Synthesis of A-Trifluoromethyl Carbonyl Compounds Phong V
DOI: 10.1002/anie.201101861 Photoredox Catalysis Photoredox Catalysis: A Mild, Operationally Simple Approach to the Synthesis of a-Trifluoromethyl Carbonyl Compounds Phong V. Pham, David A. Nagib, and David W. C. MacMillan* The unique physical and chemical advantages conferred by the CÀF bond have led to the broad exploitation of this motif throughout the pharmaceutical,[1] materials,[2] and agrochem- ical[3] sectors. In drug design, for instance, incorporation of polyfluorinated alkyl groups, such as CF3 moieties, can profoundly impact the activity, metabolic stability, lipophilic- ity, and bioavailability of lead compounds.[1,4] Not surpris- ingly, the development of methods for the production of carbonyl-based synthons bearing a-CF3 substitution has emerged as a central objective in the field of chemical synthesis. Although important recent advances have been made toward this goal, there are currently few operationally simple methods for the conversion of enolates (or enolate equivalents) to a-trifluoromethylated carbonyl motifs. Stan- dard alkylation methods are generally not productive, due to the negative polarization of the trifluoromethyl moiety, thus specially tailored reagents have been developed to furnish an trifluoromethylation of a range of enolates or enolate [5] electrophilic CF3 equivalent. Alternatively, in recent years, a equivalents [Eq. (1)]. In this context, we elected to employ set of radical (Et3B/O2) and organometallic (Rh-catalyzed) enolsilanes and silylketene acetals as suitable enolic sub- approaches have been -
Enantioselective Alkylation of Aldehydes
Angewandte Chemie International Edition: DOI: 10.1002/anie.201503789 Synthetic Methods Hot Paper German Edition: DOI: 10.1002/ange.201503789 Enantioselective a-Alkylation of Aldehydes by Photoredox Organocatalysis: Rapid Access to Pharmacophore Fragments from b- Cyanoaldehydes** Eric R. Welin, Alexander A. Warkentin, Jay C. Conrad, and David W. C. MacMillan* Abstract: The combination of photoredox catalysis and Recently, we questioned whether this dual photoredox- enamine catalysis has enabled the development of an enantio- organocatalysis platform could be translated to the asym- selective a-cyanoalkylation of aldehydes. This synergistic metric catalytic alkylation of aldehydes using a-bromo catalysis protocol allows for the coupling of two highly cyanoalkyls, a protocol that would generate b-cyanoalde- versatile yet orthogonal functionalities, allowing rapid diversi- hydes in one step. As a critical design element, we recognized fication of the oxonitrile products to a wide array of that a-bromo cyanoalkylating reagents would not be suitable medicinally relevant derivatives and heterocycles. This meth- electrophiles for most catalytic enolate addition pathways; odology has also been applied to the total synthesis of the however, the corresponding open-shell radicals, derived by lignan natural product (À)-bursehernin. one-electron reduction of a-bromonitriles, should readily undergo coupling with transiently generated chiral enamines. The enantioselective a-alkylation of carbonyl compounds In addition, the nitrile functional group offers -
Electrochemistry and Photoredox Catalysis: a Comparative Evaluation in Organic Synthesis
molecules Review Electrochemistry and Photoredox Catalysis: A Comparative Evaluation in Organic Synthesis Rik H. Verschueren and Wim M. De Borggraeve * Department of Chemistry, Molecular Design and Synthesis, KU Leuven, Celestijnenlaan 200F, box 2404, 3001 Leuven, Belgium; [email protected] * Correspondence: [email protected]; Tel.: +32-16-32-7693 Received: 30 March 2019; Accepted: 23 May 2019; Published: 5 June 2019 Abstract: This review provides an overview of synthetic transformations that have been performed by both electro- and photoredox catalysis. Both toolboxes are evaluated and compared in their ability to enable said transformations. Analogies and distinctions are formulated to obtain a better understanding in both research areas. This knowledge can be used to conceptualize new methodological strategies for either of both approaches starting from the other. It was attempted to extract key components that can be used as guidelines to refine, complement and innovate these two disciplines of organic synthesis. Keywords: electrosynthesis; electrocatalysis; photocatalysis; photochemistry; electron transfer; redox catalysis; radical chemistry; organic synthesis; green chemistry 1. Introduction Both electrochemistry as well as photoredox catalysis have gone through a recent renaissance, bringing forth a whole range of both improved and new transformations previously thought impossible. In their growth, inspiration was found in older established radical chemistry, as well as from cross-pollination between the two toolboxes. In scientific discussion, photoredox catalysis and electrochemistry are often mentioned alongside each other. Nonetheless, no review has attempted a comparative evaluation of both fields in organic synthesis. Both research areas use electrons as reagents to generate open-shell radical intermediates. Because of the similar modes of action, many transformations have been translated from electrochemical to photoredox methodology and vice versa. -
A Synergistic LUMO Lowering Strategy Using Lewis Acid Catalysis in Water to Enable Photoredox Cite This: Chem
Chemical Science View Article Online EDGE ARTICLE View Journal | View Issue A synergistic LUMO lowering strategy using Lewis acid catalysis in water to enable photoredox Cite this: Chem. Sci.,2018,9,7096 – All publication charges for this article catalytic, functionalizing C C cross-coupling of have been paid for by the Royal Society † of Chemistry styrenes Elisabeth Speckmeier, Patrick J. W. Fuchs and Kirsten Zeitler * Easily available a-carbonyl acetates serve as convenient alkyl radical source for an efficient, photocatalytic cross-coupling with a great variety of styrenes. Activation of electronically different a-acetylated acetophenone derivatives could be effected via LUMO lowering catalysis using a superior, synergistic combination of water and (water-compatible) Lewis acids. Deliberate application of fac-Ir(ppy)3 as photocatalyst to enforce an oxidative quenching cycle is crucial to the success of this (umpolung type) transformation. Mechanistic particulars of this dual catalytic coupling reaction have been studied in detail using both Stern–Volmer and cyclic voltammetry Creative Commons Attribution-NonCommercial 3.0 Unported Licence. Received 12th May 2018 experiments. As demonstrated in more than 30 examples, our water-assisted LA/photoredox Accepted 17th July 2018 catalytic activation strategy allows for excess-free, equimolar radical cross-coupling and subsequent DOI: 10.1039/c8sc02106f formal Markovnikov hydroxylation to versatile 1,4-difunctionalized products in good to excellent rsc.li/chemical-science yields. redox properties: with an oxidation potential of only 0.66 V vs. Introduction À SCE (Eox(Brc/Br ) ¼ 0.66 V) mesolytically cleaved bromide Visible light photocatalysis as a powerful tool for the selective anions can easily get oxidized to the corresponding bromine This article is licensed under a generation of radicals has given rise to numerous unprece- radicals and hence potentially may also form elemental Br2 by 8–10 dented C–C-coupling reactions in recent years.1 Especially alkyl most common photocatalysts. -
Synthesis of High Performance Phenolphthalein-Based Cardo Poly(Ether Ketone Imide)S Via Aromatic Nucleophilic Substitution Polymerization
Synthesis of high performance phenolphthalein-based cardo poly(ether ketone imide)s via aromatic nucleophilic substitution polymerization * * Jiangtao Liu, Guofei Chen , Junchao Guo, Nafeesa Mushtaq, Xingzhong Fang Ningbo Key Laboratory of Polymer Materials, Ningbo Institute of Materials Technology and Engineering, Chinese Academy of Sciences, Ningbo, Zhejiang 315201, China article info abstract Article history: A series of cardo poly(ether ketone imide)s (PEKI-C) containing large-volume bulky phthalide groups Received 13 January 2015 were prepared by aromatic nucleophilic substitution reaction of commercially available phenolphthalein Received in revised form and 4,40-bis(4-fluorophthalimido)diphenyl ether/4,40-difluorobenzophenone. The glass transition tem- 9 May 2015 peratures (T s) increased from 221 to 278 C with increasing the content of 4,40-bis(4-fluorophthalimido) Accepted 29 May 2015 g diphenyl ether moiety in the copolymerization. The 5% weight loss temperatures (T ) of PEKI-C (aee) Available online 5 June 2015 5% reached up to 472e495 C in nitrogen and 466e481 C in air. Flexible films, which could be easily cast from the polymer solutions, exhibited good mechanical properties with tensile strengths of 73e124 MPa, Keywords: e e Phenolphthalein elongations at break of 9.7 12.8%, and tensile moduli of 2.2 2.8 GPa. Their films with cut-off wave- Cardo poly(ether ketone imide)s lengths from 336 to 368 nm were transparent and essentially colorless. It is noted that the Tg, T5%, tensile Aromatic nucleophilic substitution strengths, elongations at break, and tensile moduli of PEKI-C (aee) increased with increasing the content 0 polymerization of 4,4 -bis(4-fluorophthalimido)diphenyl ether in the copolymerization. -
Merging Photoredox Catalysis with Organocatalysis
REPORTS 8. C. Resini, T. Montanari, G. Busca, J.-M. Jehng, 17. M. Salmeron, R. Schlögl, Surf. Sci. Rep. 63, 169 (2008). für Synchrotronstrahlung for support of in situ XPS I. E. Wachs, Catal. Today 99, 105 (2005). 18. T. I. T. Okpalugo, P. Papakonstantinou, H. Murphy, measurements; and M. A Smith for helpful discussions. 9. T. G. Alkhazov, E. A. Ismailov, A. Yu, A. I. Kozharov, J. McLaughlin, N. M. D. Brown, Carbon 43, 153 (2005). Supported by the CANAPE project of the 6th Framework Kinet. Katal. 19, 611 (1978). 19. L. M. Madeira, M. F. Portela, Catal. Rev. 44, 247 (2002). Programme of European Commission and the ENERCHEM 10. M. S. Kane, L. C. Kao, R. K. Mariwala, D. F. Hilscher, 20. F. Atamny et al., Mol. Phys. 76, 851 (1992). project of the Max Planck Society. H. C. Foley, Ind. Eng. Chem. Res. 35, 3319 (1996). 21. M. L. Toebes, J. M. P. van Heeswijk, J. H. Bitter, 11. M. F. R. Pereira, J. J. M. Órfão, J. L. Figueiredo, A. J. van Dillen, K. P. de Jong, Carbon 42, 307 (2004). Appl. Catal. A 218, 307 (2001). 22. A. M. Puziy, O. I. Poddubnaya, A. M. Ziatdinov, Appl. Surf. Supporting Online Material www.sciencemag.org/cgi/content/full/322/5898/73/DC1 12. J. Zhang et al., Angew. Chem. Int. Ed. 46, 7319 (2007). Sci. 252, 8036 (2006). Materials and Methods 13. G. Mestl, N. I. Maksimova, N. Keller, V. V. Roddatis, 23. S. J. Clark et al., Z. Kristallogr. 220, 567 (2005). R. Schlögl, Angew. -
Recent Advances and Future Prospects of Phthalimide Derivatives
Journal of Applied Pharmaceutical Science Vol. 6 (03), pp. 159-171, March, 2016 Available online at http://www.japsonline.com DOI: 10.7324/JAPS.2016.60330 ISSN 2231-3354 Recent Advances and Future Prospects of Phthalimide Derivatives Neelottama Kushwahaa*, Darpan Kaushikb aPranveer Singh Institute of Technology, Kanpur, India. bPrasad Institute of Technology, Jaunpur, India. ABSTRACT ARTICLE INFO Article history: Among bicyclic non-aromatic nitrogen heterocycles, phthalimides are an interesting class of compounds with a Received on: 21/05/2015 large range of applications. Phthalimide contains an imide functional group and may be considered as nitrogen Revised on: 13/09/2015 analogues of anhydrides or as diacyl derivatives of ammonia. They are lipophilic and neutral compounds and can Accepted on: 02/11/2015 therefore easily cross biological membranes in vivo and showing different pharmacological activities. In the Available online: 10/03/2016 present work compounds containing phthalimide subunit have been described as a scaffold to design new prototypes drug candidates with different biological activities and are used in different diseases as, for example Key words: AIDS, tumor, diabetes, multiple myeloma, convulsion, inflammation, pain, bacterial infection among others. Phthalimide derivatives, imide, biological activity. INTRODUCTION have served as starting materials and intermediates for the synthesis of many types of alkaloids and pharmacophores. Phthalimides possess a structural feature –CO-N(R)- CO- and an imide ring which help them to be biologically active Structure of Phthalimide and pharmaceutically useful. Phthalimides have received attention due to their androgen receptor antagonists (Sharma et Phthalimide is an imido derivative of phthalic acid. In al., 2012), anticonvulsant (Kathuria and Pathak, 2012), organic chemistry, imide is a functional group consisting of two antimicrobial (Khidre et al., 2011), hypoglycaemic (Mbarki and carbonyl groups bound to nitrogen. -
United States Patent Office Patented Sept
3,274,211 United States Patent Office Patented Sept. 20, 1966 2 cording to the process of this invention. Other ketones 3,274,211 N-ALKYLATION OF AMDES AND MEDES which may be utilized to give particular N-alkylation Louis Schmerling, Riverside, III., assignor to Universal products include methyl ethyl ketone, methyl propyl Oil Products Company, Des Plaines, Ill., a corporation ketone, methylbutyl ketone, methyl amyl ketone, methyl of Delaware hexyl ketone, methyl heptyl ketone, methyl octyl ketone, No Drawing. Filed Feb. 4, 1964, Ser. No. 342,549 etc., ethyl ethyl ketone, ethyl propyl ketone, ethyl butyl 9 Claims. (Cl. 260-326) ketone, ethyl amyl ketone, ethylhexyl ketone, ethyl hep tyl ketone, etc., propyl propyl ketone, propylbutyl ketone, This invention relates to a novel process for the N propyl amyl ketone, propylhexyl ketone, etc., butylbutyl alkylation of carboxylic acid amides and imides. More ketone, butyl amyl ketone, butyl hexyl ketone, etc. particularly, this invention relates to the reductive alkyla Other suitable but not necessarily equivalent ketones com tion of carboxylic acid amides and imides to give N prise cyclic ketones including cyclohexanone, benzophe alkylation products. none, etc., alkyl aryl ketones, alkyl cyclohexyl ketones, The alkylation products herein contemplated are par aryl aryl ketones, aryl cyclohexyl ketones, etc., including ticularly adapted to the preparation of primary amines. 15 compounds like acetophenone, ethyl phenylketone, propyl For example, N-isobutylacetamide, the reductive alkyla phenyl ketone, butyl phenyl ketone, amyl phenyl ketone, tion product of acetamide and isobutyraldehyde, is hydro etc., methyl cyclohexyl ketone, ethyl cyclohexyl ketone, lyzed to isobutylamine and acetic acid, the latter being propyl cyclohexyl ketone, butyl cyclohexyl ketone, etc. -
Photoredox-Catalyzed Silyldifluoromethylation of Silyl Enol Ethers
Photoredox-catalyzed silyldifluoromethylation of silyl enol ethers Vyacheslav I. Supranovich, Vitalij V. Levin and Alexander D. Dilman* Letter Open Access Address: Beilstein J. Org. Chem. 2020, 16, 1550–1553. N. D. Zelinsky Institute of Organic Chemistry, Leninsky prosp. 47, doi:10.3762/bjoc.16.126 119991 Moscow, Russian Federation Received: 15 April 2020 Email: Accepted: 15 June 2020 Alexander D. Dilman* - [email protected] Published: 29 June 2020 * Corresponding author This article is part of the thematic issue "Organo-fluorine chemistry V". Keywords: Guest Editor: D. O'Hagan difluoroalkylation; organofluorine compounds; photocatalysis; radical addition; silicon reagents © 2020 Supranovich et al.; licensee Beilstein-Institut. License and terms: see end of document. Abstract A method for the light-mediated fluoroalkylation of silyl enol ethers with (bromodifluoromethyl)trimethylsilane followed by a reduction of the primary products with sodium borohydride is described. An 18 W, 375 nm LED was used as the light source. The reaction is performed in the presence of a gold photocatalyst, which effects the generation of a (trimethylsilyl)difluoromethyl radical via cleavage of the carbon–bromine bond. Findings Fluorinated silicon reagents have found widespread use for the to Lewis bases and accordingly it was used as a precursor of introduction of fluorinated fragments [1-5]. Typically, these difluorocarbene, which can react with enol ethers [19,20] reagents work under strongly basic conditions required to acti- (Scheme 1). We showed that this silane could be involved in the vate inert C–Si bonds with the generation of carbanionic radical chain hydrofluoroalkylation of electron-deficient species. On the other hand, radical reactions offer different syn- alkenes, using a boron hydride as a source of hydrogen [21]. -
Visible Light Photoredox Catalysis with Transition Metal Complexes: Applications in Organic Synthesis Christopher K
Review pubs.acs.org/CR Visible Light Photoredox Catalysis with Transition Metal Complexes: Applications in Organic Synthesis Christopher K. Prier, Danica A. Rankic, and David W. C. MacMillan* Merck Center for Catalysis at Princeton University, Princeton, New Jersey 08544, United States References 5360 1. INTRODUCTION CONTENTS A fundamental aim in the field of catalysis is the development 1. Introduction 5322 of new modes of small molecule activation. One approach 2+ toward the catalytic activation of organic molecules that has 2. Photochemistry of Ru(bpy)3 5323 3. Net Reductive Reactions 5324 received much attention recently is visible light photoredox 3.1. Reduction of Electron-Poor Olefins 5324 catalysis. In a general sense, this approach relies on the ability of 3.2. Reductive Dehalogenation 5326 metal complexes and organic dyes to engage in single-electron- 3.3. Reductive Cleavage of Sulfonium and transfer (SET) processes with organic substrates upon Sulfonyl Groups 5328 photoexcitation with visible light. 3.4. Nitrogen Functional Group Reductions 5329 Many of the most commonly employed visible light 3.5. Radical Cyclizations 5330 photocatalysts are polypyridyl complexes of ruthenium and iridium, and are typified by the complex tris(2,2′-bipyridine) 3.6. Reductive Epoxide and Aziridine Opening 5331 2+ 3.7. Reduction-Labile Protecting Groups 5331 ruthenium(II), or Ru(bpy)3 (Figure 1). These complexes 4. Net Oxidative Reactions 5332 4.1. Functional Group Oxidations 5332 4.2. Oxidative Removal of the PMB Group 5334 4.3. Oxidative Biaryl Coupling 5335 4.4. Oxidative Generation of Iminium Ions 5335 4.5. Azomethine Ylide [3 + 2] Cycloadditions 5338 4.6. -
Green Radicals of Potassium Poly(Heptazine Imide) Using Light and Benzylamine† Cite This: J
Journal of Materials Chemistry A View Article Online COMMUNICATION View Journal | View Issue Green radicals of potassium poly(heptazine imide) using light and benzylamine† Cite this: J. Mater. Chem. A,2019,7, 24771 Yevheniia Markushyna,‡a Paolo Lamagni,‡abc Christian Teutloff,d c bc a a Received 28th August 2019 Jacopo Catalano, Nina Lock,* Guigang Zhang, Markus Antonietti a Accepted 7th October 2019 and Aleksandr Savateev * DOI: 10.1039/c9ta09500d rsc.li/materials-a Tinted long-lived ionic carbon nitride radicals were recently intro- poly(heptazine imides) are capable of photocharging.8 Fig. 1 duced and applied in photocatalysis and energy storage. However, the sketches the mechanism of IDEAS (Illumination-Driven reason for their higher activity in the photocatalytic reaction and Electron Accumulation in Semiconductors) – acapacious optimal conditions for generating such radicals remain vague. Herein, acronym that we propose for this process in the present Creative Commons Attribution 3.0 Unported Licence. we study the conditions for carbon nitride photocharging to achieve communication. a higher charge density and validate a convenient method to quantify On the energy scale, it can be schematically explained as the number of electrons accumulated in carbon nitride semi- follows. By absorbing a photon, potassium poly(heptazine conductors by quenching its radicals with methylviologen in the dark. imide) (K-PHI) is converted to the excited state (K-PHI*). Elec- In the presence of CO2, potassium poly(heptazine imide) (K-PHI) can tron transfer from the HOMO of the electron donor (ED) lying be charged by up to 1000 mmol of electrons per gram of the material above the valence band (VB) of K-PHI reduces K-PHI* and gives À using benzylamine as an electron donor.