Cell Cycle Checkpoint Signaling Through the ATM and ATR Kinases
Total Page:16
File Type:pdf, Size:1020Kb
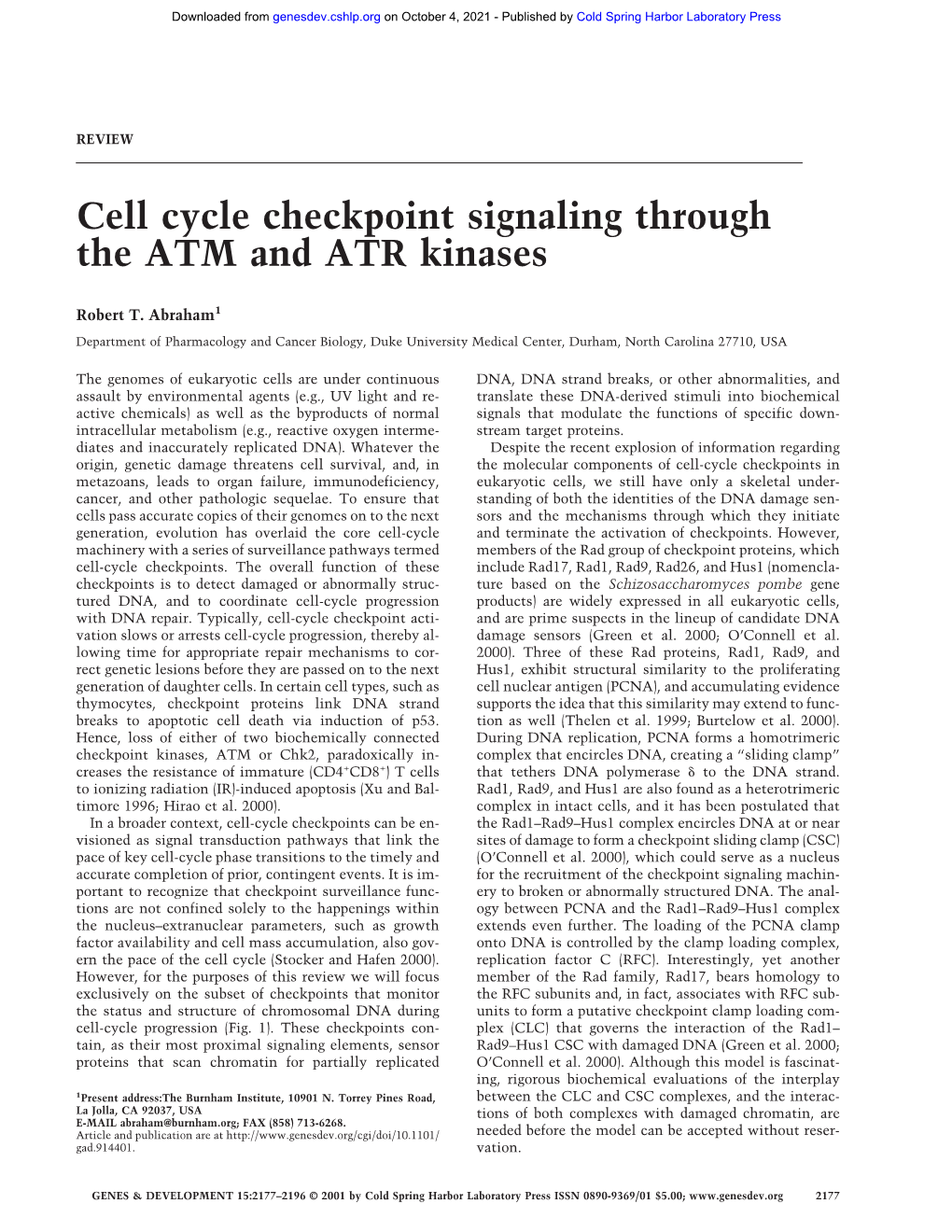
Load more
Recommended publications
-
DNA Damage Checkpoint Dynamics Drive Cell Cycle Phase Transitions
bioRxiv preprint doi: https://doi.org/10.1101/137307; this version posted August 4, 2017. The copyright holder for this preprint (which was not certified by peer review) is the author/funder, who has granted bioRxiv a license to display the preprint in perpetuity. It is made available under aCC-BY 4.0 International license. DNA damage checkpoint dynamics drive cell cycle phase transitions Hui Xiao Chao1,2, Cere E. Poovey1, Ashley A. Privette1, Gavin D. Grant3,4, Hui Yan Chao1, Jeanette G. Cook3,4, and Jeremy E. Purvis1,2,4,† 1Department of Genetics 2Curriculum for Bioinformatics and Computational Biology 3Department of Biochemistry and Biophysics 4Lineberger Comprehensive Cancer Center University of North Carolina, Chapel Hill 120 Mason Farm Road Chapel Hill, NC 27599-7264 †Corresponding Author: Jeremy Purvis Genetic Medicine Building 5061, CB#7264 120 Mason Farm Road Chapel Hill, NC 27599-7264 [email protected] ABSTRACT DNA damage checkpoints are cellular mechanisms that protect the integrity of the genome during cell cycle progression. In response to genotoxic stress, these checkpoints halt cell cycle progression until the damage is repaired, allowing cells enough time to recover from damage before resuming normal proliferation. Here, we investigate the temporal dynamics of DNA damage checkpoints in individual proliferating cells by observing cell cycle phase transitions following acute DNA damage. We find that in gap phases (G1 and G2), DNA damage triggers an abrupt halt to cell cycle progression in which the duration of arrest correlates with the severity of damage. However, cells that have already progressed beyond a proposed “commitment point” within a given cell cycle phase readily transition to the next phase, revealing a relaxation of checkpoint stringency during later stages of certain cell cycle phases. -
Cyclin-Dependent Kinase 5 Decreases in Gastric Cancer and Its
Published OnlineFirst January 21, 2015; DOI: 10.1158/1078-0432.CCR-14-1950 Biology of Human Tumors Clinical Cancer Research Cyclin-Dependent Kinase 5 Decreases in Gastric Cancer and Its Nuclear Accumulation Suppresses Gastric Tumorigenesis Longlong Cao1,2, Jiechao Zhou2, Junrong Zhang1,2, Sijin Wu3, Xintao Yang1,2, Xin Zhao2, Huifang Li2, Ming Luo1, Qian Yu1, Guangtan Lin1, Huizhong Lin1, Jianwei Xie1, Ping Li1, Xiaoqing Hu3, Chaohui Zheng1, Guojun Bu2, Yun-wu Zhang2,4, Huaxi Xu2,4,5, Yongliang Yang3, Changming Huang1, and Jie Zhang2,4 Abstract Purpose: As a cyclin-independent atypical CDK, the role of correlated with the severity of gastric cancer based on tumor CDK5 in regulating cell proliferation in gastric cancer remains and lymph node metastasis and patient 5-year fatality rate. unknown. Nuclear localization of CDK5 was found to be significantly Experimental Design: Expression of CDK5 in gastric tumor decreased in tumor tissues and gastric cancer cell lines, and paired adjacent noncancerous tissues from 437 patients was whereas exogenously expression of nucleus-targeted CDK5 measured by Western blotting, immunohistochemistry, and real- inhibited the proliferation and xenograft implantation of time PCR. The subcellular translocation of CDK5 was monitored gastric cancer cells. Treatment with the small molecule during gastric cancer cell proliferation. The role of nuclear CDK5 NS-0011, which increases CDK5 accumulation in the nucleus, in gastric cancer tumorigenic proliferation and ex vivo xenografts suppressed both cancer cell proliferation and xenograft was explored. Furthermore, by screening for compounds in the tumorigenesis. PubChem database that disrupt CDK5 association with its nu- Conclusions: Our results suggest that low CDK5 expression is clear export facilitator, we identified a small molecular (NS-0011) associated with poor overall survival in patients with gastric that inhibits gastric cancer cell growth. -
ATR Pathway Inhibition Is Synthetically Lethal in Cancer Cells with ERCC1 Deficiency
Published OnlineFirst March 24, 2014; DOI: 10.1158/0008-5472.CAN-13-3229 Cancer Therapeutics, Targets, and Chemical Biology Research ATR Pathway Inhibition Is Synthetically Lethal in Cancer Cells with ERCC1 Deficiency Kareem N. Mohni, Gina M. Kavanaugh, and David Cortez Abstract The DNA damage response kinase ATR and its effector kinase CHEK1 are required for cancer cells to survive oncogene-induced replication stress. ATR inhibitors exhibit synthetic lethal interactions, with deficiencies in the DNA damage response enzymes ATM and XRCC1 and with overexpression of the cell cycle kinase cyclin E. Here, we report a systematic screen to identify synthetic lethal interactions with ATR pathway–targeted drugs, rationalized by their predicted therapeutic utility in the oncology clinic. We found that reduced function in the ATR pathway itself provided the strongest synthetic lethal interaction. In addition, we found that loss of the structure-specific endonuclease ERCC1-XPF (ERCC4) is synthetic lethal with ATR pathway inhibitors. ERCC1- deficient cells exhibited elevated levels of DNA damage, which was increased further by ATR inhibition. When treated with ATR or CHEK1 inhibitors, ERCC1-deficient cells were arrested in S-phase and failed to complete cell-cycle transit even after drug removal. Notably, triple-negative breast cancer cells and non–small cell lung cancer cells depleted of ERCC1 exhibited increased sensitivity to ATR pathway–targeted drugs. Overall, we concluded that ATR pathway–targeted drugs may offer particular utility in cancers with reduced ATR pathway function or reduced levels of ERCC4 activity. Cancer Res; 74(10); 1–11. Ó2014 AACR. Introduction repair pathway such as homologous recombination or post- – Replicating DNA is sensitive to a wide array of endogenous replicative repair to remove the PARP DNA complexes (7). -
NBN Gene Analysis and It's Impact on Breast Cancer
Journal of Medical Systems (2019) 43: 270 https://doi.org/10.1007/s10916-019-1328-z IMAGE & SIGNAL PROCESSING NBN Gene Analysis and it’s Impact on Breast Cancer P. Nithya1 & A. ChandraSekar1 Received: 8 March 2019 /Accepted: 7 May 2019 /Published online: 5 July 2019 # Springer Science+Business Media, LLC, part of Springer Nature 2019 Abstract Single Nucleotide Polymorphism (SNP) researches have become essential in finding out the congenital relationship of structural deviations with quantitative traits, heritable diseases and physical responsiveness to different medicines. NBN is a protein coding gene (Breast Cancer); Nibrin is used to fix and rebuild the body from damages caused because of strand breaks (both singular and double) associated with protein nibrin. NBN gene was retrieved from dbSNP/NCBI database and investigated using computational SNP analysis tools. The encrypted region in SNPs (exonal SNPs) were analyzed using software tools, SIFT, Provean, Polyphen, INPS, SNAP and Phd-SNP. The 3’ends of SNPs in un-translated region were also investigated to determine the impact of binding. The association of NBN gene polymorphism leads to several diseases was studied. Four SNPs were predicted to be highly damaged in coding regions which are responsible for the diseases such as, Aplastic Anemia, Nijmegan breakage syndrome, Microsephaly normal intelligence, immune deficiency and hereditary cancer predisposing syndrome (clivar). The present study will be helpful in finding the suitable drugs in future for various diseases especially for breast cancer. Keywords NBN . Single nucleotide polymorphism . Double strand breaks . nsSNP . Associated diseases Introduction NBN has a more complex structure due to its interaction with large proteins formed from the ATM gene which is NBN (Nibrin) is a protein coding gene, it is also known as highly essential in identifying damaged strands of DNA NBS1, Cell cycle regulatory Protein P95, is situated on and facilitating their repair [1]. -
Metabolic Checkpoints in Cancer Cell Cycle
City University of New York (CUNY) CUNY Academic Works All Dissertations, Theses, and Capstone Projects Dissertations, Theses, and Capstone Projects 2-2014 Metabolic Checkpoints in Cancer Cell Cycle Mahesh Saqcena Graduate Center, City University of New York How does access to this work benefit ou?y Let us know! More information about this work at: https://academicworks.cuny.edu/gc_etds/106 Discover additional works at: https://academicworks.cuny.edu This work is made publicly available by the City University of New York (CUNY). Contact: [email protected] METABOLIC CHECKPOINTS IN CANCER CELL CYCLE By Mahesh Saqcena A dissertation submitted to the Graduate Faculty in Biochemistry in partial fulfillment of the requirements for the degree of Doctor of Philosophy, The City University of New York 2014 i 2014 Mahesh Saqcena All Rights Reserved ii This manuscript has been read and accepted for the Graduate Faculty in Biochemistry in satisfaction of the dissertation requirement for the degree of Doctor of Philosophy. Date Dr. David A. Foster (Chair of Examining Committee) Date Dr. Edward J. Kennelly (Executive Officer) Dr. Mitchell Goldfarb (Hunter College) Dr. Paul Feinstein (Hunter College) Dr. Richard Kolesnick (Sloan Kettering Institute) Dr. Frederick R. Cross (Rockefeller University) (Supervisory Committee) THE CITY UNIVERSITY OF NEW YORK iii Abstract METABOLIC CHECKPOINTS IN CANCER CELL CYCLE by Mahesh Saqcena Advisor: Dr. David A. Foster Growth factors (GFs) as well as nutrient sufficiency regulate cell division in metazoans. The vast majority of mutations that contribute to cancer are in genes that regulate progression through the G1 phase of the cell cycle. A key regulatory site in G1 is the growth factor-dependent Restriction Point (R), where cells get permissive signals to divide. -
Mitosis Vs. Meiosis
Mitosis vs. Meiosis In order for organisms to continue growing and/or replace cells that are dead or beyond repair, cells must replicate, or make identical copies of themselves. In order to do this and maintain the proper number of chromosomes, the cells of eukaryotes must undergo mitosis to divide up their DNA. The dividing of the DNA ensures that both the “old” cell (parent cell) and the “new” cells (daughter cells) have the same genetic makeup and both will be diploid, or containing the same number of chromosomes as the parent cell. For reproduction of an organism to occur, the original parent cell will undergo Meiosis to create 4 new daughter cells with a slightly different genetic makeup in order to ensure genetic diversity when fertilization occurs. The four daughter cells will be haploid, or containing half the number of chromosomes as the parent cell. The difference between the two processes is that mitosis occurs in non-reproductive cells, or somatic cells, and meiosis occurs in the cells that participate in sexual reproduction, or germ cells. The Somatic Cell Cycle (Mitosis) The somatic cell cycle consists of 3 phases: interphase, m phase, and cytokinesis. 1. Interphase: Interphase is considered the non-dividing phase of the cell cycle. It is not a part of the actual process of mitosis, but it readies the cell for mitosis. It is made up of 3 sub-phases: • G1 Phase: In G1, the cell is growing. In most organisms, the majority of the cell’s life span is spent in G1. • S Phase: In each human somatic cell, there are 23 pairs of chromosomes; one chromosome comes from the mother and one comes from the father. -
Kinetochores, Microtubules, and Spindle Assembly Checkpoint
Review Joined at the hip: kinetochores, microtubules, and spindle assembly checkpoint signaling 1 1,2,3 Carlos Sacristan and Geert J.P.L. Kops 1 Molecular Cancer Research, University Medical Center Utrecht, 3584 CG Utrecht, The Netherlands 2 Center for Molecular Medicine, University Medical Center Utrecht, 3584 CG Utrecht, The Netherlands 3 Cancer Genomics Netherlands, University Medical Center Utrecht, 3584 CG Utrecht, The Netherlands Error-free chromosome segregation relies on stable and cell division. The messenger is the SAC (also known as connections between kinetochores and spindle microtu- the mitotic checkpoint) (Figure 1). bules. The spindle assembly checkpoint (SAC) monitors The transition to anaphase is triggered by the E3 ubiqui- such connections and relays their absence to the cell tin ligase APC/C, which tags inhibitors of mitotic exit cycle machinery to delay cell division. The molecular (CYCLIN B) and of sister chromatid disjunction (SECURIN) network at kinetochores that is responsible for microtu- for proteasomal degradation [2]. The SAC has a one-track bule binding is integrated with the core components mind, inhibiting APC/C as long as incorrectly attached of the SAC signaling system. Molecular-mechanistic chromosomes persist. It goes about this in the most straight- understanding of how the SAC is coupled to the kineto- forward way possible: it assembles a direct and diffusible chore–microtubule interface has advanced significantly inhibitor of APC/C at kinetochores that are not connected in recent years. The latest insights not only provide a to spindle microtubules. This inhibitor is named the striking view of the dynamics and regulation of SAC mitotic checkpoint complex (MCC) (Figure 1). -
Supplementary Table S1. Correlation Between the Mutant P53-Interacting Partners and PTTG3P, PTTG1 and PTTG2, Based on Data from Starbase V3.0 Database
Supplementary Table S1. Correlation between the mutant p53-interacting partners and PTTG3P, PTTG1 and PTTG2, based on data from StarBase v3.0 database. PTTG3P PTTG1 PTTG2 Gene ID Coefficient-R p-value Coefficient-R p-value Coefficient-R p-value NF-YA ENSG00000001167 −0.077 8.59e-2 −0.210 2.09e-6 −0.122 6.23e-3 NF-YB ENSG00000120837 0.176 7.12e-5 0.227 2.82e-7 0.094 3.59e-2 NF-YC ENSG00000066136 0.124 5.45e-3 0.124 5.40e-3 0.051 2.51e-1 Sp1 ENSG00000185591 −0.014 7.50e-1 −0.201 5.82e-6 −0.072 1.07e-1 Ets-1 ENSG00000134954 −0.096 3.14e-2 −0.257 4.83e-9 0.034 4.46e-1 VDR ENSG00000111424 −0.091 4.10e-2 −0.216 1.03e-6 0.014 7.48e-1 SREBP-2 ENSG00000198911 −0.064 1.53e-1 −0.147 9.27e-4 −0.073 1.01e-1 TopBP1 ENSG00000163781 0.067 1.36e-1 0.051 2.57e-1 −0.020 6.57e-1 Pin1 ENSG00000127445 0.250 1.40e-8 0.571 9.56e-45 0.187 2.52e-5 MRE11 ENSG00000020922 0.063 1.56e-1 −0.007 8.81e-1 −0.024 5.93e-1 PML ENSG00000140464 0.072 1.05e-1 0.217 9.36e-7 0.166 1.85e-4 p63 ENSG00000073282 −0.120 7.04e-3 −0.283 1.08e-10 −0.198 7.71e-6 p73 ENSG00000078900 0.104 2.03e-2 0.258 4.67e-9 0.097 3.02e-2 Supplementary Table S2. -
Mutations in the Nijmegen Breakage Syndrome Gene (NBS1) in Childhood Acute Lymphoblastic Leukemia (ALL)1
[CANCER RESEARCH 61, 3570–3572, May 1, 2001] Advances in Brief Mutations in the Nijmegen Breakage Syndrome Gene (NBS1) in Childhood Acute Lymphoblastic Leukemia (ALL)1 Raymonda Varon, Andre´Reis,2 Gu¨nter Henze, Hagen Graf v. Einsiedel, Karl Sperling, and Karlheinz Seeger Institute of Human Genetics [R. V., A. R., K. Sp.] and Department of Pediatric Oncology/Hematology [G. H., H. G. v. E., K. Se.], Charite´, Humboldt-University, 13353 Berlin, Germany, and Molecular Genetics and Gene Mapping Centre, Max-Delbrueck-Centre, 13092 Berlin, Germany [A. R.] Abstract protein—a FHA and a BRCT, both spanning the first 200 amino acids of nibrin (9)—that are also present in a number of other proteins The Nijmegen Breakage Syndrome (NBS) is a rare autosomal recessive involved in the cell cycle control (10, 11). On the basis of epidemi- disorder associated with immune deficiency, chromosome fragility, and ological data, it has been suggested that NBS heterozygotes also have increased susceptibility to lymphoid malignancies. The aim of the present an elevated cancer risk (12) similar to AT or other syndromes asso- study was to elucidate the potential role of the gene mutated in NBS (NBS1) in the pathogenesis and disease progression of childhood acute ciated with immune deficiencies (4). The findings that the ATM gene lymphoblastic leukemia (ALL). Samples from 47 children with first re- is involved in the pathogenesis of B-CLL (13, 14) and T-cell prolym- lapse of ALL were analyzed for mutations in all 16 exons of the NBS1 phocytic leukemia (15) as well as in breast cancer (16) implicate its gene, and in 7 of them (14.9%), four novel amino acid substitutions were role as a tumor suppressor gene. -
Identification of the Interactors of Human Nibrin (NBN) and of Its 26 Kda and 70 Kda Fragments Arising from the NBN 657Del5 Founder Mutation
RESEARCH ARTICLE Identification of the Interactors of Human Nibrin (NBN) and of Its 26 kDa and 70 kDa Fragments Arising from the NBN 657del5 Founder Mutation Domenica Cilli1., Cristiana Mirasole2., Rosa Pennisi1, Valeria Pallotta2, Angelo D’Alessandro2, Antonio Antoccia1,3, Lello Zolla2, Paolo Ascenzi3,4, Alessandra di Masi1,3* 1. Department of Science, Roma Tre University, Rome, Italy, 2. Department of Ecological and Biological Sciences, University of Tuscia, Viterbo, Italy, 3. Istituto Nazionale Biostrutture e Biosistemi – Consorzio Interuniversitario, Rome, Italy, 4. Interdepartmental Laboratory for Electron Microscopy, Roma Tre University, Rome, Italy *[email protected] . These authors contributed equally to this work. OPEN ACCESS Citation: Cilli D, Mirasole C, Pennisi R, Pallotta V, Abstract D’Alessandro A, et al. (2014) Identification of the Interactors of Human Nibrin (NBN) and of Its 26 Nibrin (also named NBN or NBS1) is a component of the MRE11/RAD50/NBN kDa and 70 kDa Fragments Arising from the NBN complex, which is involved in early steps of DNA double strand breaks sensing and 657del5 Founder Mutation. PLoS ONE 9(12): e114651. doi:10.1371/journal.pone.0114651 repair. Mutations within the NBN gene are responsible for the Nijmegen breakage Editor: Sue Cotterill, St. Georges University of syndrome (NBS). The 90% of NBS patients are homozygous for the 657del5 London, United Kingdom mutation, which determines the synthesis of two truncated proteins of 26 kDa (p26) Received: October 28, 2013 and 70 kDa (p70). Here, HEK293 cells have been exploited to transiently express Accepted: November 12, 2014 either the full-length NBN protein or the p26 or p70 fragments, followed by affinity Published: December 8, 2014 chromatography enrichment of the eluates. -
Interferon-A-Induced G1 Phase Arrest Through Up-Regulated Expression of CDK Inhibitors, P19ink4d and P21cip1 in Mouse Macrophages
Oncogene (1998) 16, 2075 ± 2086 1998 Stockton Press All rights reserved 0950 ± 9232/98 $12.00 http://www.stockton-press.co.uk/onc Interferon-a-induced G1 phase arrest through up-regulated expression of CDK inhibitors, p19Ink4D and p21Cip1 in mouse macrophages Masaaki Matsuoka, Kenzaburo Tani and Shigetaka Asano Department of Hematology and Oncology, Institute of Medical Science, University of Tokyo, 4-6-1 Shirokanedai, Minato-ku, Tokyo 108, Japan The mechanism of cell cycle arrest induced by interferon-a dependent kinases or cdks (reviewed by Sherr, 1993). (IFN-a) was analysed using a mouse macrophage cell line, D-type cyclins (Lew et al., 1991; Matsushime et al., 1991) BAC1.2F5A. IFN-a added in media before mid-G1 and cyclin E (Ko et al., 1991; Lew et al., 1991) govern the prohibited cells from entering S phase. The blockage of G1/S transition in association with their proper G1/S transition was associated with diminuition of both physiological partners, cdk4 (Matsushime et al., 1992) cyclin D1/cdk4- and cyclin E/cdk2-associated kinase or cdk6 (Meyerson and Harlow, 1994), and cdk2 (Dulic et activities. G1 cyclin-associated kinase activities were al., 1992; Ko et al., 1992), respectively. When cells enter down-regulated quickly after the addition of IFN-a. Cells G1 phase with mitogenic stimuli, the synthesis and the treated with IFN-a contained excess amounts of cdk active complex formation of D-type cyclins and cdk4 are inhibitors which down-regulated G1 cyclin/cdk-associated induced in early to middle G1 phase, and their complex kinase activities in the proliferating cells and this action formation and activities reach maximal levels at late G1 was counteracted by exogenously-supplied recombinant (Matsushime et al., 1992, 1994). -
Targeting the WEE1 Kinase As a Molecular Targeted Therapy for Gastric Cancer
www.impactjournals.com/oncotarget/ Oncotarget, Vol. 7, No. 31 Research Paper Targeting the WEE1 kinase as a molecular targeted therapy for gastric cancer Hye-Young Kim1,2, Yunhee Cho1,3, HyeokGu Kang1,3, Ye-Seal Yim1,3, Seok-Jun Kim1,3, Jaewhan Song2, Kyung-Hee Chun1,3 1Department of Biochemistry & Molecular Biology, Yonsei University College of Medicine, Seodaemun-gu, Seoul 03722, Korea 2Department of Biochemistry, College of Life Science and Biotechnology, Seodaemun-gu, Seoul 03722, Korea 3Brain Korea 21 PlusProject for Medical Science, Yonsei University, Seodaemun-gu, Seoul 03722, Korea Correspondence to: Kyung-Hee Chun, email: [email protected] Keywords: WEE1, AZD1775 (MK-1775), 5-FU, Paclitaxel, gastric cancer Received: September 07, 2015 Accepted: May 28, 2016 Published: June 23, 2016 ABSTRACT Wee1 is a member of the Serine/Threonine protein kinase family and is a key regulator of cell cycle progression. It has been known that WEE1 is highly expressed and has oncogenic functions in various cancers, but it is not yet studied in gastric cancers. In this study, we investigated the oncogenic role and therapeutic potency of targeting WEE1 in gastric cancer. At first, higher expression levels of WEE1 with lower survival probability were determined in stage 4 gastric cancer patients or male patients with accompanied lymph node metastasis. To determine the function of WEE1 in gastric cancer cells, we determined that WEE1 ablation decreased the proliferation, migration, and invasion, while overexpression of WEE1 increased these effects in gastric cancer cells. We also validated the clinical application of WEE1 targeting by a small molecule, AZD1775 (MK-1775), which is a WEE1 specific inhibitor undergoing clinical trials.