All-Electric Quantum Point Contact Spin-Polarizer P
Total Page:16
File Type:pdf, Size:1020Kb
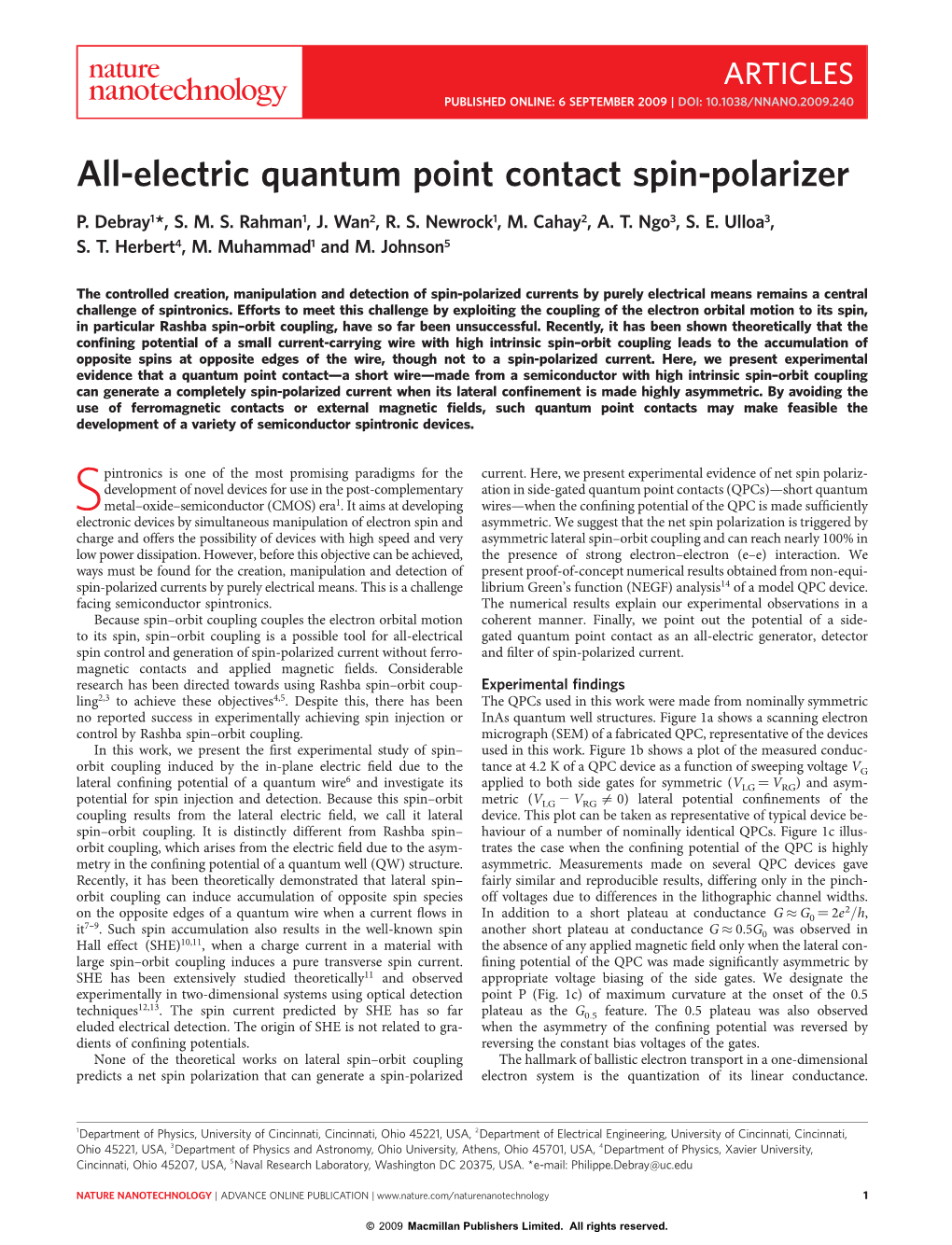
Load more
Recommended publications
-
Quantum Dots
Quantum Dots www.nano4me.org © 2018 The Pennsylvania State University Quantum Dots 1 Outline • Introduction • Quantum Confinement • QD Synthesis – Colloidal Methods – Epitaxial Growth • Applications – Biological – Light Emitters – Additional Applications www.nano4me.org © 2018 The Pennsylvania State University Quantum Dots 2 Introduction Definition: • Quantum dots (QD) are nanoparticles/structures that exhibit 3 dimensional quantum confinement, which leads to many unique optical and transport properties. Lin-Wang Wang, National Energy Research Scientific Computing Center at Lawrence Berkeley National Laboratory. <http://www.nersc.gov> GaAs Quantum dot containing just 465 atoms. www.nano4me.org © 2018 The Pennsylvania State University Quantum Dots 3 Introduction • Quantum dots are usually regarded as semiconductors by definition. • Similar behavior is observed in some metals. Therefore, in some cases it may be acceptable to speak about metal quantum dots. • Typically, quantum dots are composed of groups II-VI, III-V, and IV-VI materials. • QDs are bandgap tunable by size which means their optical and electrical properties can be engineered to meet specific applications. www.nano4me.org © 2018 The Pennsylvania State University Quantum Dots 4 Quantum Confinement Definition: • Quantum Confinement is the spatial confinement of electron-hole pairs (excitons) in one or more dimensions within a material. – 1D confinement: Quantum Wells – 2D confinement: Quantum Wire – 3D confinement: Quantum Dot • Quantum confinement is more prominent in semiconductors because they have an energy gap in their electronic band structure. • Metals do not have a bandgap, so quantum size effects are less prevalent. Quantum confinement is only observed at dimensions below 2 nm. www.nano4me.org © 2018 The Pennsylvania State University Quantum Dots 5 Quantum Confinement • Recall that when atoms are brought together in a bulk material the number of energy states increases substantially to form nearly continuous bands of states. -
Interaction Effects in a Microscopic Quantum Wire Model with Strong OPEN ACCESS Spin–Orbit Interaction
PAPER • OPEN ACCESS Related content - Correlation effects in two-dimensional Interaction effects in a microscopic quantum wire topological insulators M Hohenadler and F F Assaad model with strong spin–orbit interaction - Charge dynamics of the antiferromagnetically ordered Mott insulator To cite this article: G W Winkler et al 2017 New J. Phys. 19 063009 Xing-Jie Han, Yu Liu, Zhi-Yuan Liu et al. - One-dimensional Fermi liquids J Voit View the article online for updates and enhancements. This content was downloaded from IP address 131.211.105.193 on 19/02/2018 at 15:46 New J. Phys. 19 (2017) 063009 https://doi.org/10.1088/1367-2630/aa7027 PAPER Interaction effects in a microscopic quantum wire model with strong OPEN ACCESS spin–orbit interaction RECEIVED 16 January 2017 G W Winkler1,2, M Ganahl2,3, D Schuricht4, H G Evertz2,5 and S Andergassen6,7 REVISED 1 Theoretical Physics and Station Q Zurich, ETH Zurich, 8093 Zurich, Switzerland 9 April 2017 2 Institute of Theoretical and Computational Physics, Graz University of Technology, A-8010 Graz, Austria ACCEPTED FOR PUBLICATION 3 Perimeter Institute for Theoretical Physics, Waterloo, Ontario N2L 2Y5, Canada 28 April 2017 4 Institute for Theoretical Physics, Center for Extreme Matter and Emergent Phenomena, Utrecht University, Princetonplein 5, 3584 CE PUBLISHED Utrecht, The Netherlands 5 June 2017 5 Kavli Institute for Theoretical Physics, University of California, Santa Barbara, CA 93106, United States of America 6 Institute for Theoretical Physics and Center for Quantum Science, Universität Tübingen, Auf der Morgenstelle 14, D-72076 Tübingen, Original content from this Germany work may be used under 7 Faculty of Physics, University of Vienna, Boltzmanngasse 5, A-1090 Vienna, Austria the terms of the Creative Commons Attribution 3.0 E-mail: [email protected] licence. -
Quantized Transmission in an Asymmetrically Biased Quantum Point Contact
Linköping University | Department of Physics, Chemistry and Biology Master’s thesis, 30 hp | Master’s programme in Physics and Nanoscience Autumn term 2016 | LITH-IFM-A-EX—16/3274--SE Quantized Transmission in an Asymmetrically Biased Quantum Point Contact Erik Johansson Examinator, Magnus Johansson Supervisors, Irina Yakimenko & Karl-Fredrik Berggren Avdelning, institution Datum Division, Department Date Theoretical Physics 2016-11-07 Department of Physics, Chemistry and Biology Linköping University, SE-581 83 Linköping, Sweden Språk Rapporttyp ISBN Language Report category Svenska/Swedish Licentiatavhandling ISRN: LITH-IFM-A-EX--16/3274--SE Engelska/English Examensarbete _________________________________________________________________ C-uppsats D-uppsats Serietitel och serienummer ISSN ________________ Övrig rapport Title of series, numbering ______________________________ _____________ URL för elektronisk version Titel Title Quantized Transmission in an Asymmetrically Biased Quantum Point Contact Författare Author Erik Johansson Sammanfattning Abstract In this project work we have studied how a two-dimensional electron gas (2DEG) in a GaAs/AlGaAs semiconductor heterostructure can be locally confined down to a narrow bottleneck constriction called a quantum point contact (QPC) and form an artificial quantum wire using a split-gate technique by application of negative bias voltages. The electron transport through the QPC and how asymmetric loading of bias voltages affects the nature of quantized conductance were studied. The basis is -
A Practical Phase Gate for Producing Bell Violations in Majorana Wires
PHYSICAL REVIEW X 6, 021005 (2016) A Practical Phase Gate for Producing Bell Violations in Majorana Wires David J. Clarke, Jay D. Sau, and Sankar Das Sarma Department of Physics, Condensed Matter Theory Center, University of Maryland, College Park, Maryland 20742, USA and Joint Quantum Institute, University of Maryland, College Park, Maryland 20742, USA (Received 9 October 2015; published 8 April 2016) Carrying out fault-tolerant topological quantum computation using non-Abelian anyons (e.g., Majorana zero modes) is currently an important goal of worldwide experimental efforts. However, the Gottesman- Knill theorem [1] holds that if a system can only perform a certain subset of available quantum operations (i.e., operations from the Clifford group) in addition to the preparation and detection of qubit states in the computational basis, then that system is insufficient for universal quantum computation. Indeed, any measurement results in such a system could be reproduced within a local hidden variable theory, so there is no need for a quantum-mechanical explanation and therefore no possibility of quantum speedup [2]. Unfortunately, Clifford operations are precisely the ones available through braiding and measurement in systems supporting non-Abelian Majorana zero modes, which are otherwise an excellent candidate for topologically protected quantum computation. In order to move beyond the classically simulable subspace, an additional phase gate is required. This phase gate allows the system to violate the Bell-like Clauser- Horne-Shimony-Holt (CHSH) inequality that would constrain a local hidden variable theory. In this article, we introduce a new type of phase gate for the already-existing semiconductor-based Majorana wire systems and demonstrate how this phase gate may be benchmarked using CHSH measurements. -
Transport Properties of Clean Quantum Point Contacts
Home Search Collections Journals About Contact us My IOPscience Transport properties of clean quantum point contacts This article has been downloaded from IOPscience. Please scroll down to see the full text article. 2011 New J. Phys. 13 113006 (http://iopscience.iop.org/1367-2630/13/11/113006) View the table of contents for this issue, or go to the journal homepage for more Download details: IP Address: 188.155.208.225 The article was downloaded on 05/11/2011 at 07:08 Please note that terms and conditions apply. New Journal of Physics The open–access journal for physics Transport properties of clean quantum point contacts CRossler¨ 1, S Baer, E de Wiljes, P-L Ardelt, T Ihn, K Ensslin, C Reichl and W Wegscheider Solid State Physics Laboratory, ETH Zurich, 8093 Zurich, Switzerland E-mail: [email protected] New Journal of Physics 13 (2011) 113006 (16pp) Received 6 June 2011 Published 3 November 2011 Online at http://www.njp.org/ doi:10.1088/1367-2630/13/11/113006 Abstract. Quantum point contacts are fundamental building blocks for mesoscopic transport experiments and play an important role in recent interference and fractional quantum Hall experiments. However, it is unclear how electron–electron interactions and the random disorder potential influence the confinement potential and give rise to phenomena such as the mysterious 0.7 anomaly. Novel growth techniques of AlX Ga1 X As heterostructures for high- mobility two-dimensional electron gases enable− us to investigate quantum point contacts with a strongly suppressed disorder potential. These clean quantum point contacts indeed show transport features that are obscured by disorder in standard samples. -
Carbon Nanotubes……
UNIT VI Engineering Materials CHAPTER-19 Nanomaterials and Their Applications “We are placing bets on things we can do uniquely well. First, new ways to power the world. Second, molecular medicine. And third, nanotechnology.” - Jeff Immelt, CEO, General Electric What is Nanotech? Nano Technology – Art and science of manipulating atoms and molecules to create new systems, materials, and devices. Nanomeasurement – Size Nanomanipulation – Building from the bottom up. Size Matters How Big is a Nano? – Nano = 1 billionth;100,000 x’s smaller than the diameter of a human hair. Examples of Nanoscale – A cubic micron of water contains about 90 billion atoms. A micron is one thousandth of a millimeter, and a thousand times larger than a nanometer. – Another way to visualize a nanometer: 1 inch = 25,400,000 nanometers Size Matters in context to Nano Size SIGNIFICANCE OF THE NANOSCALE ➢The science dealing with the materials of the nanoworld is an extension of the existing science into the nanoscale or a recasting of existing sciences using a newer, more modern terms. ➢Nanoscience is based on the fact that the properties of materials change with the function of physical dimensions of the materials. ➢The properties of the materials are different at nanolevel due to two main reasons: increased surface area and quantum mechanical effect. Surface Area ➢For a sphere of radius r, the surface area and its volume can be given as ➢Thus, we find that when the given volume is divided into smaller parts, surface area increases. Quantum Confinement Effect ➢ At reduced dimensions, they are said to be either a quantum well, a quantum dot, or a quantum wire. -
2.4.2. Calculation of the Density of States in 1, 2 and 3 Dimensions
2.4.2. Calculation of the density of states in 1, 2 and 3 dimensions We will here postulate that the density of electrons in k–space is constant and equals the physical length of the sample divided by 2p and that for each dimension. The number of states between k and k + dk in 3, 2 and 1 dimension then equals: dN L dN L dN L (2.4.8) 3D = 2( )3 4p k 2, 2D = 2( )2 , 2p k 1D = 2( ) dk 2p dk 2p dk 2p We now assume that the electrons in a semiconductor are close to a band minimum, Emin and can be described as free particles with a constant effective mass, or: h2k 2 (2.4.9) E(k) = Emin + 8p 2m* Elimination of k using the E(k) relation above then yields the desired density of states functions, namely: (2.4.10) dN3D 8p 2 *3 / 2 gc,3D = = m E - Emin ,for E ³ Emin dE h3 for a three-dimensional semiconductor, * (2.4.11) dN2D 4p m gc,2D = = ,for E ³ Emin dE h2 For a two-dimensional semiconductor such as a quantum well in which particles are confined to a plane, and (2.4.12) dN 2p m* 1 g = 1D = ,for E ³ E c,1D 2 min dE h E - Emin For a one-dimensional semiconductor such as a quantum wire in which particles are confined along a line. An example of the density of states in 3, 2 and 1 dimension is shown in the figure below: Review of Modern Physics 5 1.6E+21 ) 1.4E+21 -1 1.2E+21 eV -3 1.0E+21 8.0E+20 6.0E+20 4.0E+20 Density (cm 2.0E+20 0.0E+00 0 20 40 60 80 Energy (meV) Figure 2.4.2 Density of states per unit volume and energy for a 3-D semiconductor (blue curve), a 10 nm quantum well with infinite barriers (red curve) and a 10 nm by 10 * nm quantum wire with infinite barriers (green curve). -
The 0.7-Anomaly in Quantum Point Contact; Many-Body Or Single-Electron Effect?
The 0.7-anomaly in quantum point contact; many-body or single-electron effect? Tadeusz Figielski Institute of Physics, Polish Academy of Sciences, Warsaw, Poland E-mail: [email protected] Apart from usual quantization steps on the ballistic conductance of quasi-one-dimensional conductor, an additional plateau-like feature appears at a fraction of about 0.7 below the first conductance step in GaAs- based quantum point contacts (QPCs). Despite a tremendous amount of research on this anomalous feature, its origin remains still unclear. Here, an unique model of this anomaly is proposed relied on fundamental principles of quantum mechanics. It is noticed that just after opening a quasi-1D conducting channel in the QPC a single electron travels the channel at a time, and such electron can be – in principle – observed. The act of observation destroys superposition of spin states, in which the electron otherwise exists, and this suppresses their quantum interference. It is shown that the QPC-conductance is then reduced by a factor of 0.74. “Visibility” of electron is enhanced if the electron spends some time in the channel due to resonant transmission. Electron’s resonance can also explain an unusual temperature behavior of the anomaly as well as its recently discovered feature: oscillatory modulation as a function of the channel length and electrostatic potential. A recipe for experimental verification of the model is given. PACS numbers: 73.63.-b; 73.63. 1. Introduction Short, narrow constrictions connecting two reservoirs of two-dimensional electron gas (2DEG) in semiconductor heterostructures, called quantum point contacts, exhibit at low temperature a quantization of 2 the ballistic conductance in units of G0 = 2e /h (e and h are the elementary charge and the Planck’s constant, respectively, and the factor 2 arises from spin degeneracy). -
Quantum Wells/Dots and Nanowires for Optoelectronicdevice
����������� ��������� �� ��� ���� ������� �� �������������� ���������� ������������ ��� ����������������� �� ���� � � ���� ���� ������� ���������� � ��������� ��� �������������� ������ ������������ ������� �� ��� ����� �� ���������� ��������� ����������� ��� ���������� �������� ���������� ��������� ��������� The Australian National University Research School of Physical Sciences and Engineering QuantumQuantum Wells/DotsWells/Dots andand NanowiresNanowires forfor OptoelectronicOptoelectronic DeviceDevice ApplicationsApplications Michael Q. Gao, H. Hoe Tan, Chennupati Jagadish ([email protected]) Department of Electronic Materials Engineering Research School of Physical Sciences and Engineering The Australian National University, Canberra, Australia Department of Electronic Materials Engineering The Australian National University Research School of Physical Sciences and Engineering The Group Few people are missing (M. Buda, Y. Kim, Q. Gao, J. Wong-Leung, H. Hattori) Department of Electronic Materials Engineering The Australian National University Research School of Physical Sciences and Engineering Overview • Introduction • Growth of Quantum Dots by Metal Organic Chemical Vapour Deposition (MOCVD) • Towards Quantum Well/Dot Photonic Integrated Circuits – Intermixing (QW/QD) by ion implantation ¾ Quantum Well/Dot Lasers ¾ Quantum Well/Dot Infrared Photodetectors (QDIPs) • Carrier lifetime Modification by Ion Implantation for untra-fast detectors and THz emitters • Nanowires • Summary Department of Electronic Materials Engineering The -
Quantum Wires and Waveguides Formed in Graphene by Strain
Quantum wires and waveguides formed in graphene by strain Y. Wu,y D. Zhai,z C. Pan,y B. Cheng,y T. Taniguchi,{ K. Watanabe,{ N. Sandler,∗,z and M. Bockrath∗,x yDepartment of Physics and Astronomy, University of California, Riverside, California 92521, USA zDepartment of Physics and Astronomy, Ohio University, Athens, Ohio 45701-2979, USA {Advanced Materials Laboratory, National Institute for Materials Science, Tsukuba, Ibaraki 305-0044, Japan xDepartment of Physics, The Ohio State University, Columbus, OH 43210, USA E-mail: [email protected]; [email protected] Abstract Confinement of electrons in graphene to make devices has proven to be a chal- lenging task. Electrostatic methods fail because of Klein tunneling, while etching into nanoribbons requires extreme control of edge terminations, and bottom-up approaches are limited in size to a few nm. Fortunately, its mechanical flexibility raises the possibil- ity of using strain to alter graphene's properties and create novel straintronic devices. arXiv:1804.00207v1 [cond-mat.mes-hall] 31 Mar 2018 Here, we report transport studies of nanowires created by linearly-shaped strained re- gions resulting from individual folds formed by layer transfer onto hexagonal boron nitride. Conductance measurements across the folds reveal Coulomb blockade signa- tures, indicating confined charges within these structures, which act as quantum dots. Along folds, we observe sharp features in traverse resistivity measurements, attributed 1 to an amplification of the dot conductance modulations by a resistance bridge incor- porating the device. Our data indicates ballistic transport up to ∼1 µm along the folds. Calculations using the Dirac model including strain are consistent with mea- sured bound state energies and predict the existence of valley-polarized currents. -
Thermoelectric Transport in Quantum Point Contacts and Chaotic Cavities: Thermal Effects and Fluctuations Adel Abbout
Thermoelectric transport in quantum point contacts and chaotic cavities: thermal effects and fluctuations Adel Abbout To cite this version: Adel Abbout. Thermoelectric transport in quantum point contacts and chaotic cavities: thermal effects and fluctuations. Mesoscopic Systems and Quantum Hall Effect [cond-mat.mes-hall]. Université Pierre et Marie Curie - Paris VI, 2011. English. tel-00793816 HAL Id: tel-00793816 https://tel.archives-ouvertes.fr/tel-00793816 Submitted on 23 Feb 2013 HAL is a multi-disciplinary open access L’archive ouverte pluridisciplinaire HAL, est archive for the deposit and dissemination of sci- destinée au dépôt et à la diffusion de documents entific research documents, whether they are pub- scientifiques de niveau recherche, publiés ou non, lished or not. The documents may come from émanant des établissements d’enseignement et de teaching and research institutions in France or recherche français ou étrangers, des laboratoires abroad, or from public or private research centers. publics ou privés. Université de Paris 6 Années 2008-2011 Transport thermoélectrique dans des contacts quantiques ponctuels et de cavités chaotiques : Effets thermiques et fluctuations. Service de Physique de l’État Condensé CEA Saclay 21 Décembre 2011 Adel Abbout Directeur de thèse : Jean-Louis PICHARD Jury : Doucot BENOIT Klaus FRAHM Jean-Louis PICHARD (Directeur de thèse) Marc SANQUER Dima SHEPELYANSKY (rapporteur) Dietmar WEINMANN (rapporteur) 2 . 3 . To my parents 4 Acknowledgement I would like to thank my thesis advisor Jean Louis Pichard for all the work achieved together. I record my gratitude to him for his supervision, advice, guidance and specially his presence during all this years. His patience and kindness are well appreciated. -
Nonlinear Spectra of Spinons and Holons in Short Gaas Quantum Wires
ARTICLE Received 18 Nov 2015 | Accepted 2 Aug 2016 | Published 15 Sep 2016 DOI: 10.1038/ncomms12784 OPEN Nonlinear spectra of spinons and holons in short GaAs quantum wires M. Moreno1, C.J.B. Ford1, Y. Jin1, J.P. Griffiths1, I. Farrer1,w, G.A.C. Jones1, D.A. Ritchie1, O. Tsyplyatyev2,w & A.J. Schofield2 One-dimensional electronic fluids are peculiar conducting systems, where the fundamental role of interactions leads to exotic, emergent phenomena, such as spin-charge (spinon-holon) separation. The distinct low-energy properties of these 1D metals are successfully described within the theory of linear Luttinger liquids, but the challenging task of describing their high-energy nonlinear properties has long remained elusive. Recently, novel theoretical approaches accounting for nonlinearity have been developed, yet the rich phenomenology that they predict remains barely explored experimentally. Here, we probe the nonlinear spectral characteristics of short GaAs quantum wires by tunnelling spectroscopy, using an advanced device consisting of 6000 wires. We find evidence for the existence of an inverted (spinon) shadow band in the main region of the particle sector, one of the central predictions of the new nonlinear theories. A (holon) band with reduced effective mass is clearly visible in the particle sector at high energies. 1 Cavendish Laboratory, University of Cambridge, JJ Thomson Avenue, Cambridge CB3 0HE, UK. 2 School of Physics and Astronomy, University of Birmingham, Edgbaston, Birmingham B15 2TT, UK. w Present addresses: Department of Electronic & Electrical Engineering, University of Sheffield, Mappin Street, Sheffield S1 3JD, UK (I.F.); Institut fu¨r Theoretische Physik, Universita¨t Frankfurt, Max-von-Laue Strasse 1, 60438 Frankfurt, Germany (O.T.).