Tandem Riboswitches Form a Natural Boolean Logic Gate to Control
Total Page:16
File Type:pdf, Size:1020Kb
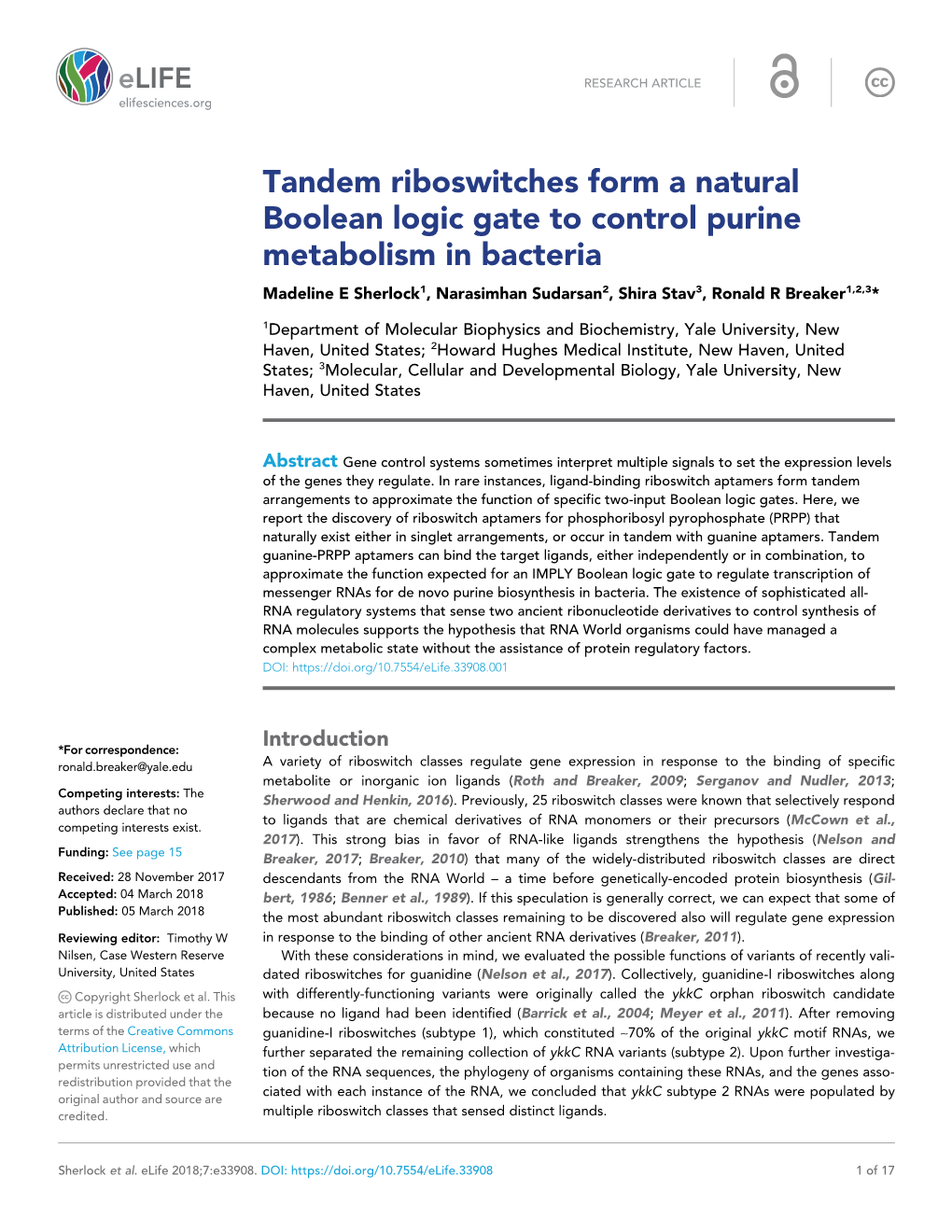
Load more
Recommended publications
-
Guanine Riboswitch Variants from Mesoplasma Florum Selectively Recognize 2-Deoxyguanosine
Guanine riboswitch variants from Mesoplasma florum selectively recognize 2-deoxyguanosine Jane N. Kim†, Adam Roth‡, and Ronald R. Breaker†‡§¶ †Department of Molecular, Cellular, and Developmental Biology, §Department of Molecular Biophysics and Biochemistry, and ‡Howard Hughes Medical Institute, Yale University, P.O. Box 208103, New Haven, CT 06520-8103 Edited by Jeffrey W. Roberts, Cornell University, Ithaca, NY, and approved August 21, 2007 (received for review June 22, 2007) Several mRNA aptamers have been identified in Mesoplasma florum witch aptamers for the modified nucleobase 7-aminomethyl-7- that have sequence and structural features resembling those of deazaguanine have been identified that require as few as 34 guanine and adenine riboswitches. Two features distinguish these nucleotides to form a selective and high-affinity binding pocket RNAs from established purine-sensing riboswitches. All possess short- (31). Moreover, there are three classes of aptamers for S- ened hairpin-loop sequences expected to alter tertiary contacts adenosylmethionine (SAM) (23, 24, 32) whose representatives are known to be critical for aptamer folding. The RNAs also carry nucle- more rare in bacteria than the SAM-I class of riboswitches com- otide changes in the core of each aptamer that otherwise is strictly monly found in Gram-positive bacteria (33–35). These findings conserved in guanine and adenine riboswitches. Some aptamers indicate that a far greater diversity of metabolite-sensing ribos- retain the ability to selectively bind guanine or adenine despite these witches exists that might be difficult for existing search strategies to mutations. However, one variant type exhibits selective and high- definitively identify and classify. affinity binding of 2-deoxyguanosine, which is consistent with its One possibility is that some organisms will have recently evolved occurrence in the 5 untranslated region of an operon containing riboswitch classes with aptamers that are unique in architecture or ribonucleotide reductase genes. -
Modeling and Searching for Non-Coding RNA
Modeling and Searching for Non-Coding RNA W.L. Ruzzo http://www.cs.washington.edu/homes/ruzzo Outline • Why RNA? • Examples of RNA biology • Computational Challenges – Modeling – Search – Inference The “Central Dogma” DNA RNA Protein gene Protein DNA (chromosome) RNA (messenger) cell “Classical” RNAs • mRNA • tRNA • rRNA • snRNA (small nuclear - spli cing) • snoRNA (small nucleolar - guides for t/rRNA modifications) • RNAseP (tRNA maturation; ribozyme in bacteria) • SRP (signal recognition particle; co-translational targeting of proteins to membranes) • telomerases Non-coding RNA • Messenger RNA - codes for proteins • Non-coding RNA - all the rest – Before, say, mid 1990’s, 1-2 dozen known (critically important, but narrow roles: e.g. tRNA) • Since mid 90’s dramatic discoveries – Regulation, transport, stability/degradation – E.g. “microRNA”: ≈ 100’s in humans • By some estimates, ncRNA >> mRNA RNA Secondary Structure: RNA makes helices too U C A A Base pairs G C C G A A U G C U A C G C G A U G C A A CA AU RNA on the Rise • In humans – more RNA- than DNA-binding proteins? – much more conserved DNA than coding – MUCH more transcribed DNA than coding • In bacteria – regulation of MANY genes involves RNA – dozens of classes & thousands of new examples in just last 5 years Human Predictions • Evofold – S Pedersen, G Bejerano, A Siepel, K Rosenbloom, K Lindblad-Toh, ES Lander, J Kent, W Miller, D Haussler, "Identification and classification of conserved RNA secondary structures in the human genome." PLoS Comput. Biol., 2, #4 (2006) e33. – 48,479 candidates (~70% FDR?) • RNAz – S Washietl, IL Hofacker, M Lukasser, A H殳 tenhofer, PF Stadler, "Mapping of conserved RNA secondary structures predicts thousands of functional noncoding RNAs in the human genome." Nat. -
Developing a Riboswitch-Mediated Regulatory System for Metabolic Flux Control in Thermophilic Bacillus Methanolicus
International Journal of Molecular Sciences Article Developing a Riboswitch-Mediated Regulatory System for Metabolic Flux Control in Thermophilic Bacillus methanolicus Marta Irla , Sigrid Hakvåg and Trygve Brautaset * Department of Biotechnology and Food Sciences, Norwegian University of Science and Technology, 7034 Trondheim, Norway; [email protected] (M.I.); [email protected] (S.H.) * Correspondence: [email protected]; Tel.: +47-73593315 Abstract: Genome-wide transcriptomic data obtained in RNA-seq experiments can serve as a re- liable source for identification of novel regulatory elements such as riboswitches and promoters. Riboswitches are parts of the 50 untranslated region of mRNA molecules that can specifically bind various metabolites and control gene expression. For that reason, they have become an attractive tool for engineering biological systems, especially for the regulation of metabolic fluxes in industrial microorganisms. Promoters in the genomes of prokaryotes are located upstream of transcription start sites and their sequences are easily identifiable based on the primary transcriptome data. Bacillus methanolicus MGA3 is a candidate for use as an industrial workhorse in methanol-based biopro- cesses and its metabolism has been studied in systems biology approaches in recent years, including transcriptome characterization through RNA-seq. Here, we identify a putative lysine riboswitch in B. methanolicus, and test and characterize it. We also select and experimentally verify 10 putative B. methanolicus-derived promoters differing in their predicted strength and present their functionality in combination with the lysine riboswitch. We further explore the potential of a B. subtilis-derived purine riboswitch for regulation of gene expression in the thermophilic B. methanolicus, establishing a Citation: Irla, M.; Hakvåg, S.; novel tool for inducible gene expression in this bacterium. -
From Genotype to Phenotype: Inferring Relationships Between Microbial Traits and Genomic Components
From genotype to phenotype: inferring relationships between microbial traits and genomic components Inaugural-Dissertation zur Erlangung des Doktorgrades der Mathematisch-Naturwissenschaftlichen Fakult¨at der Heinrich-Heine-Universit¨atD¨usseldorf vorgelegt von Aaron Weimann aus Oberhausen D¨usseldorf,29.08.16 aus dem Institut f¨urInformatik der Heinrich-Heine-Universit¨atD¨usseldorf Gedruckt mit der Genehmigung der Mathemathisch-Naturwissenschaftlichen Fakult¨atder Heinrich-Heine-Universit¨atD¨usseldorf Referent: Prof. Dr. Alice C. McHardy Koreferent: Prof. Dr. Martin J. Lercher Tag der m¨undlichen Pr¨ufung: 24.02.17 Selbststandigkeitserkl¨ arung¨ Hiermit erkl¨areich, dass ich die vorliegende Dissertation eigenst¨andigund ohne fremde Hilfe angefertig habe. Arbeiten Dritter wurden entsprechend zitiert. Diese Dissertation wurde bisher in dieser oder ¨ahnlicher Form noch bei keiner anderen Institution eingereicht. Ich habe bisher keine erfolglosen Promotionsversuche un- ternommen. D¨usseldorf,den . ... ... ... (Aaron Weimann) Statement of authorship I hereby certify that this dissertation is the result of my own work. No other person's work has been used without due acknowledgement. This dissertation has not been submitted in the same or similar form to other institutions. I have not previously failed a doctoral examination procedure. Summary Bacteria live in almost any imaginable environment, from the most extreme envi- ronments (e.g. in hydrothermal vents) to the bovine and human gastrointestinal tract. By adapting to such diverse environments, they have developed a large arsenal of enzymes involved in a wide variety of biochemical reactions. While some such enzymes support our digestion or can be used for the optimization of biotechnological processes, others may be harmful { e.g. mediating the roles of bacteria in human diseases. -
Isobaculum Melis Gen. Nov., Sp. Nov., a Carnobacterium-Like Organism
International Journal of Systematic and Evolutionary Microbiology (2002), 52, 207–210 Printed in Great Britain Isobaculum melis gen. nov., sp. nov., a NOTE Carnobacterium-like organism isolated from the intestine of a badger 1 School of Food Biosciences, Matthew D. Collins,1 Roger A. Hutson,1 Geoffrey Foster,2 Enevold Falsen3 Whiteknights, University 4 of Reading, Reading and Norbert Weiss RG6 6AP, UK 2 SAC Veterinary Science Author for correspondence: Matthew D. Collins. Tel: j44 118 935 7000. Fax: j44 118 926 7917. Division, Drummondhill, e-mail: m.d.collins!reading.ac.uk Inverness, UK 3 Culture Collection, Department of Clinical Phenotypic and phylogenetic studies were performed on a hitherto Bacteriology, University of undescribed facultatively anaerobic, catalase-negative, Gram-positive rod- Go$ teborg, Go$ teborg T Sweden shaped organism, strain M577-94 , isolated from the small intestine of a dead badger. It resembled carnobacteria in terms of its long-chain cellular fatty acid 4 Deutsche Sammlung von Mikroorganismen und composition, but differed markedly from the latter in possessing a cell-wall Zellkulturen GmbH, murein based on L-lysine (type L-Lys–L-Thr–Gly). Comparative 16S rRNA gene Braunschweig, Germany sequencing showed that the unknown bacterium represents a new line closely related to, albeit distinct from, the genera Carnobacterium and Desemzia.On the basis of phylogenetic and phenotypic evidence, it is proposed that strain M577-94T be classified as Isobaculum melis gen. nov., sp. nov. The type strain of Isobaculum melis is CCUG 37660T (l DSM 13760T). Keywords: Isobaculum melis, 16S rRNA, taxonomy, phylogeny The genus Carnobacterium was proposed as the genus known non-spore-forming rod-shaped bacterium, accommodating the species Lactobacillus divergens isolated from a badger, which somewhat resembles and Lactobacillus piscicola and a number of atypical carnobacteria. -
An Internal Residue Mimicking the Purine Ligand Vanessa Delfosse1, Patricia Bouchard1, Eric Bonneau1, Pierre Dagenais1, Jean-Franc¸ Ois Lemay2, Daniel A
Published online 18 December 2009 Nucleic Acids Research, 2010, Vol. 38, No. 6 2057–2068 doi:10.1093/nar/gkp1080 Riboswitch structure: an internal residue mimicking the purine ligand Vanessa Delfosse1, Patricia Bouchard1, Eric Bonneau1, Pierre Dagenais1, Jean-Franc¸ ois Lemay2, Daniel A. Lafontaine2,* and Pascale Legault1,* 1De´ partement de Biochimie, Universite´ de Montre´ al, C.P. 6128, Succursale Centre-Ville, Montre´ al, Que´ bec, H3C 3J7 and 2Groupe ARN/RNA Group, De´ partement de Biologie, Faculte´ des Sciences, Universite´ de Sherbrooke, Sherbrooke, Que´ bec, J1K 2R1, Canada Received September 16, 2009; Revised November 3, 2009; Accepted November 4, 2009 ABSTRACT modulate gene expression in response to the concentration level of their cognate ligand (1–3). They are positioned The adenine and guanine riboswitches regulate in the 50-UTR of bacterial mRNA and are composed of gene expression in response to their purine ligand. two overlapping domains: a purine-binding aptamer X-ray structures of the aptamer moiety of these domain and a downstream expression platform. Binding riboswitches are characterized by a compact fold of the ligand stabilizes the formation of a compact in which the ligand forms a Watson–Crick base aptamer structure, which prevents the formation of pair with residue 65. Phylogenetic analyses an alternate structure and thereby modulates gene revealed a strict restriction at position 39 of the expression. aptamer that prevents the G39–C65 and A39–U65 Depending on the expression platform composition, combinations, and mutational studies indicate that gene expression control takes place at the levels of tran- aptamers with these sequence combinations are scription or translation, and ligand binding, either posi- tively or negatively, regulates gene expression (1–3). -
Bioinformatic Analysis of Riboswitch Structures Uncovers Variant Classes
Bioinformatic analysis of riboswitch structures PNAS PLUS uncovers variant classes with altered ligand specificity Zasha Weinberga,1,2, James W. Nelsonb,1,3, Christina E. Lünseb,4, Madeline E. Sherlockc, and Ronald R. Breakera,b,c,5 aHoward Hughes Medical Institute, Yale University, New Haven, CT 06520; bDepartment of Molecular, Cellular and Developmental Biology, Yale University, New Haven, CT 06520; and cDepartment of Molecular Biophysics and Biochemistry, Yale University, New Haven, CT 06520 Contributed by Ronald R. Breaker, February 2, 2017 (sent for review December 8, 2016; reviewed by Robert T. Batey and Elena Rivas) Riboswitches are RNAs that form complex, folded structures that Several variant riboswitches share a number of characteristics selectively bind small molecules or ions. As with certain groups of that could have been exploited in a bioinformatic search for such protein enzymes and receptors, some riboswitch classes have RNAs. We chose to apply three important properties common to evolved to change their ligand specificity. We developed a pro- the guanine/adenine (15) and c-di-GMP-I/c-AMP-GMP (13, 14) cedure to systematically analyze known riboswitch classes to find riboswitch sets, among other variants. The first of these proper- additional variants that have altered their ligand specificity. This ties is that some variant riboswitches with altered ligand speci- approach uses multiple-sequence alignments, atomic-resolution ficity will remain somewhat close in both sequence and structure structural information, and riboswitch gene associations. Among to the predominant or “parent” class. For example, the initial the discoveries are unique variants of the guanine riboswitch class collections of representatives for guanine (23) and c-di-GMP-I ′ that most tightly bind the nucleoside 2 -deoxyguanosine. -
L NOTE Human Vagina Matthew D
international Journal of Systematic Bacteriology (1 999), 49, 1 125-1 128 Printed in Great Britain Aerococcus christensenii sp. nov., f rom the L NOTE human vagina Matthew D. Collins,’ Mar Rodriguez Jovita,’ Roger A. Hutson,’ Maria Ohlen2and Enevold Faisen’ Author for correspondence: Matthew D. Collins. Tel: +44 118 935 7000. Fax: +44 118 926 7917 e-mail : [email protected] 1 Department of Food Phenotypic and phylogenetic studies were performed on two strains of a Science and Technology, hitherto undescribed Aerococcus-like organism isolated from the human University of Reading, Reading RG6 6AP. UK vagina. Comparative 16s rRNA gene sequencing studies demonstratedthat the unknown strains constitute a new subline within the genus Aerococcus. The 2 Culture Collection, Department of Clinical unknown bacterium was readily distinguished from the two currently Bacteriology, University of recognized Aerococcus species, Aerococcus viridans and Aerococcus urinae, by Gsteborg, 5-41346 biochemical tests and electrophoretic analysis of whole-cell proteins. On the Gbteborg, Sweden basis of phylogenetic and phenotypic evidence, it is proposed that the unknown bacterium be classified as Aerococcus christenseniisp. nov. The type strain of A. christenseniiis CCUG 2883IT. Keywords: Aerococcus christensenii sp. nov., phylogeny, taxonomy, 16s rRNA - The genus Aerococcus until recently contained a single UW06T (= CCUG 2883 lT), were submitted to the species, Aerococcus viridans (Williams et al., 1953). Culture Collection of the University of Goteborg by This species is found in a wide range of environments L. K. Rabe and S. L. Hillier (University of Washing- and, although considered saprophytic, has been shown ton, Seattle, USA) for identification. Both strains were to cause disease in lobsters and to be associated, albeit isolated from vaginal sources and tentatively identified rarely, with human infections, e.g. -
Synthetic Ligands for Preq1 Riboswitches Provide Structural and Mechanistic Insights Into Targeting RNA Tertiary Structure
ARTICLE https://doi.org/10.1038/s41467-019-09493-3 OPEN Synthetic ligands for PreQ1 riboswitches provide structural and mechanistic insights into targeting RNA tertiary structure Colleen M. Connelly1, Tomoyuki Numata2,3, Robert E. Boer1, Michelle H. Moon1, Ranu S. Sinniah1, Joseph J. Barchi1, Adrian R. Ferré-D’Amaré 2 & John S. Schneekloth Jr. 1 1234567890():,; Riboswitches are naturally occurring RNA aptamers that regulate gene expression by binding to specific small molecules. Riboswitches control the expression of essential bacterial genes and are important models for RNA-small molecule recognition. Here, we report the discovery of a class of synthetic small molecules that bind to PreQ1 riboswitch aptamers. These molecules bind specifically and reversibly to the aptamers with high affinity and induce a conformational change. Furthermore, the ligands modulate riboswitch activity through tran- scriptional termination despite no obvious chemical similarity to the cognate ligand. X-ray crystallographic studies reveal that the ligands share a binding site with the cognate ligand but make different contacts. Finally, alteration of the chemical structure of the ligand causes changes in the mode of RNA binding and affects regulatory function. Thus, target- and structure-based approaches can be used to identify and understand the mechanism of synthetic ligands that bind to and regulate complex, folded RNAs. 1 Chemical Biology Laboratory, National Cancer Institute, Frederick, MD 21701, USA. 2 Biochemistry and Biophysics Center, National Heart, Lung and Blood Institute, Bethesda, MD 20892, USA. 3 Biomedical Research Institute, National Institute of Advanced Industrial Science and Technology (AIST), Tsukuba, Ibaraki 305-8566, Japan. These authors contributed equally: Colleen M. -
Loss of Conserved Noncoding Rnas in Genomes of Bacterial Endosymbionts
GBE Loss of Conserved Noncoding RNAs in Genomes of Bacterial Endosymbionts Dorota Matelska1, Malgorzata Kurkowska1, Elzbieta Purta1, Janusz M. Bujnicki1,2,*and Stanislaw Dunin-Horkawicz1,* 1Laboratory of Bioinformatics and Protein Engineering, International Institute of Molecular and Cell Biology, Warsaw, Poland 2Laboratory of Structural Bioinformatics, Institute of Molecular Biology and Biotechnology, Adam Mickiewicz University, Poznan, Poland *Corresponding author: E-mail: [email protected]; [email protected]. Accepted: January 11, 2016 Abstract The genomes of intracellular symbiotic or pathogenic bacteria, such as of Buchnera, Mycoplasma,andRickettsia, are typically smaller compared with their free-living counterparts. Here we showed that noncoding RNA (ncRNA) families, which are conserved in free- living bacteria, frequently could not be detected by computational methods in the small genomes. Statistical tests demonstrated that their absence is not an artifact of low GC content or small deletions in these small genomes, and thus it was indicative of an independent loss of ncRNAs in different endosymbiotic lineages. By analyzing the synteny (conservation of gene order) between the reduced and nonreduced genomes, we revealed instances of protein-coding genes that were preserved in the reduced genomes but lost cis-regulatory elements. We found that the loss of cis-regulatory ncRNA sequences, which regulate the expression of cognate protein-coding genes, is characterized by the reduction of secondary structure formation propensity, GC content, and length of the corresponding genomic regions. Key words: endosymbionts, noncoding RNA loss, covariance models, Rfam. Introduction The length of fully sequenced bacterial genomes ranges from 2001), and chromosomes undergo rearrangements (Belda 110 kb (Bennett and Moran 2013)to15Mb(Han et al. -
Core Requirements of the Adenine Riboswitch Aptamer for Ligand Binding
JOBNAME: RNA 13#3 2007 PAGE: 1 OUTPUT: Wednesday February 7 07:09:20 2007 csh/RNA/131630/rna1420 Downloaded from rnajournal.cshlp.org on September 29, 2021 - Published by Cold Spring Harbor Laboratory Press Core requirements of the adenine riboswitch aptamer for ligand binding JEAN-FRANCxOIS LEMAY and DANIEL A. LAFONTAINE De´partement de biologie, Faculte´ des sciences, Universite´ de Sherbrooke, Que´bec, J1K 2R1, Canada ABSTRACT The adenine riboswitch aptamer, the A box, positively regulates gene expression upon adenine binding. To provide insight into structure–function relationships, important for the adenine riboswitch aptamer, we have created alignments for six aptamer sequences that reveal the core requirements. In addition, 2-aminopurine (2AP) binding studies have been used to test the consensus sequence derived from the alignment. Overall, the consensus secondary structure is consistent with 2AP binding studies. However, a position in the core, previously identified as variable, shows restriction in nucleotide sequence. Further- more, this restriction is found to be related with the ligand specificity of the riboswitch. The implications of this relationship for the riboswitch gene regulation mechanism are discussed. Keywords: riboswitch; RNA structure; RNA folding; aptamer; molecular recognition; fluorescence spectroscopy INTRODUCTION 2003), glucosamine-6-phosphate (Winkler et al. 2004), glycine (Mandal et al. 2004), lysine (Grundy et al. 2003; Riboswitches are regulatory elements found predominantly Sudarsan et al. 2003b), intracellular magnesium (Cromie in 59-untranslated regions of messenger RNAs (mRNAs). et al. 2006), S-adenosylmethionine (Epshtein et al. 2003; These genetic switches are highly structured domains that McDaniel et al. 2003; Winkler et al. 2003), and thiamine can bind cellular metabolites and regulate the expression of pyrophosphate (Mironov et al. -
Gene Expression Control by Bacillus Anthracis Purine Riboswitches
Downloaded from rnajournal.cshlp.org on September 30, 2021 - Published by Cold Spring Harbor Laboratory Press Kirchner and Schneider 1 Gene expression control by Bacillus anthracis purine riboswitches Marion Kirchner* and Sabine Schneider* Center for Integrated Protein Science at the Department of Chemistry Technische Universität München, Lichtenbergstrasse 4, 85748 Garching, Germany * To whom correspondence should be addressed. Tel: +49 89 289 13336; Fax: +49 89 289 13363; Email: [email protected]; [email protected] Running Title: Purine riboswitches of Bacillus anthracis Keywords (max 6): purine riboswitches, Bacillus anthracis, purine biosynthesis, nucleobase salvage, nucleotide metabolism ABSTRACT In all kingdoms of life, cellular replication relies on the presence of nucleosides and nucleotides, the building blocks of nucleic acids and the main source of energy. In bacteria, the availability of metabolites sometimes directly regulates the expression of enzymes and proteins involved in purine salvage, biosynthesis and uptake through riboswitches. Riboswitches are located in bacterial mRNAs and can control gene expression by conformational changes in response to ligand binding. We have established an inverse reporter gene system in Bacillus subtilis that allows us to monitor riboswitch-controlled gene expression. We used it to investigate the activity of five potential purine riboswitches from B. anthracis in response to different purines and pyrimidines. Furthermore, in vitro studies on the aptamer domains of the riboswitches reveal their variation in guanine binding affinity ranging from nM to µM. These data do not only provide insight into metabolite sensing but can also aid to engineer artificial cell regulatory systems. Downloaded from rnajournal.cshlp.org on September 30, 2021 - Published by Cold Spring Harbor Laboratory Press Kirchner and Schneider 2 INTRODUCTION Riboswitches are structural elements in the 5’ untranslated region of mRNAs, which consist of an aptamer domain and an expression platform (Fig.