Network Analysis of Down Syndrome and SARS-Cov-2 Identifies Risk And
Total Page:16
File Type:pdf, Size:1020Kb
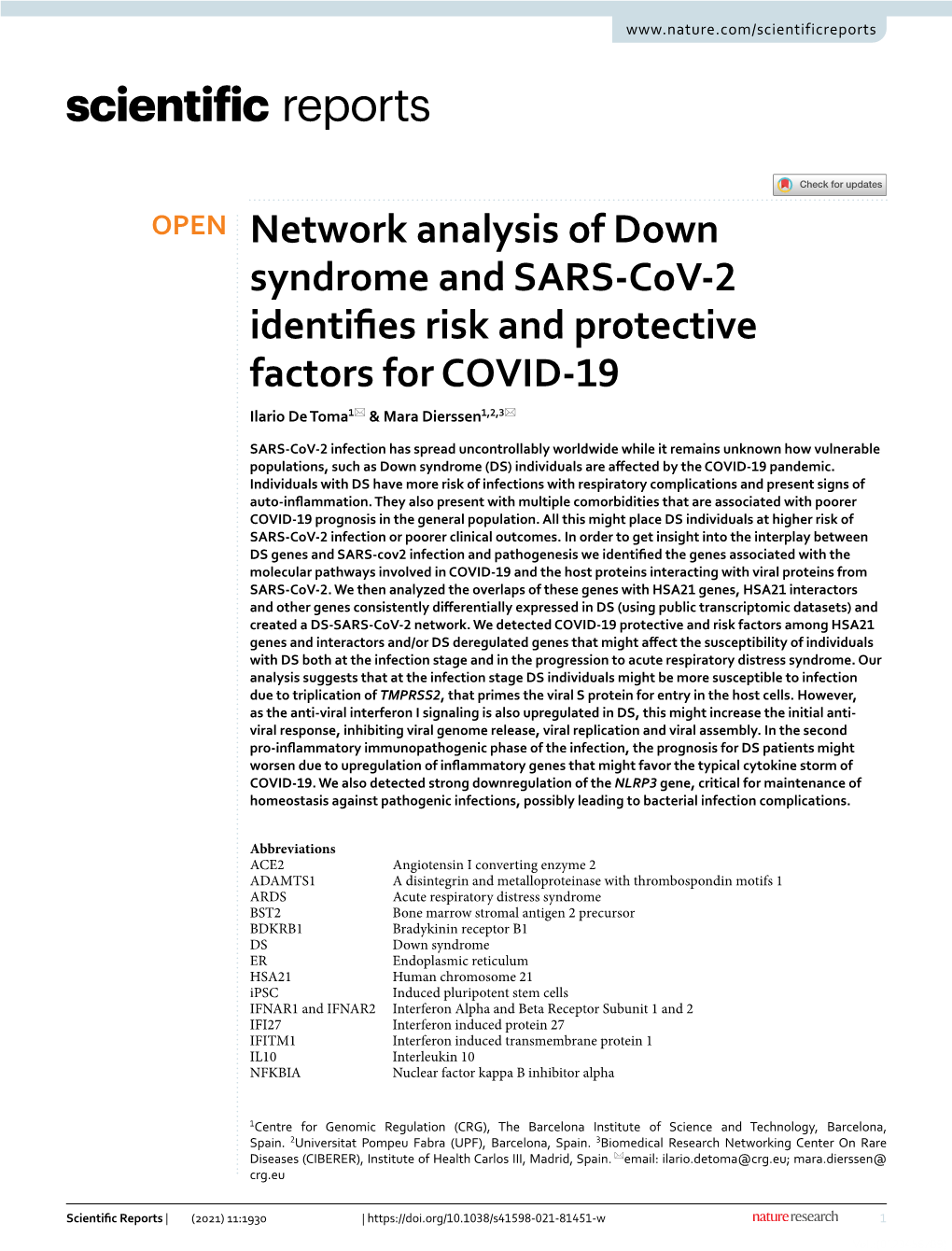
Load more
Recommended publications
-
(12) Patent Application Publication (10) Pub. No.: US 2012/0070450 A1 Ishikawa Et Al
US 20120070450A1 (19) United States (12) Patent Application Publication (10) Pub. No.: US 2012/0070450 A1 Ishikawa et al. (43) Pub. Date: Mar. 22, 2012 (54) LEUKEMA STEM CELLMARKERS Publication Classification (51) Int. Cl. A 6LX 39/395 (2006.01) (75) Inventors: Fumihiko Ishikawa, Kanagawa CI2O I/68 (2006.01) (JP): Osamu Ohara, Kanagawa GOIN 2L/64 (2006.01) (JP); Yoriko Saito, Kanagawa (JP); A6IP35/02 (2006.01) Hiroshi Kitamura, Kanagawa (JP); C40B 30/04 (2006.01) Atsushi Hijikata, Kanagawa (JP); A63L/7088 (2006.01) Hidetoshi Ozawa, Kanagawa (JP); C07K 6/8 (2006.01) Leonard D. Shultz, Bar Harbor, C7H 2L/00 (2006.01) A6II 35/12 (2006.01) ME (US) CI2N 5/078 (2010.01) (52) U.S. Cl. .................. 424/173.1; 424/178.1; 424/93.7: (73) Assignee: RIKEN, Wako-shi (JP) 435/6.14; 435/723; 435/375; 506/9: 514/44 A: 530/389.6; 530/391.7:536/24.5 (57) ABSTRACT (21) Appl. No.: 13/258,993 The invention provides a test method for predicting the initial onset or a recurrence of acute myeloid leukemia (AML) com PCT Fled: prising (1) measuring the expression level of human leukemic (22) Mar. 24, 2010 stem cell (LSC) marker genes in a biological sample collected from a Subject for a transcription product or translation prod uct of the gene as an analyte and (2) comparing the expression (86) PCT NO.: PCT/UP2010/0551.31 level with a reference value; an LSC-targeting therapeutic agent for AML capable of Suppressing the expression of a S371 (c)(1), gene selected from among LSC marker genes or a Substance (2), (4) Date: Dec. -
Activation of ATM Depends on Chromatin Interactions Occurring Before Induction of DNA Damage
LETTERS Activation of ATM depends on chromatin interactions occurring before induction of DNA damage Yong-Chul Kim1,6, Gabi Gerlitz1,6, Takashi Furusawa1, Frédéric Catez1,5, Andre Nussenzweig2, Kyu-Seon Oh3, Kenneth H. Kraemer3, Yosef Shiloh4 and Michael Bustin1,7 Efficient and correct responses to double-stranded breaks the KAP-1 protein9. The local and global changes in chromatin organiza- (DSB) in chromosomal DNA are crucial for maintaining genomic tion facilitate recruitment of damage-response proteins and remodelling stability and preventing chromosomal alterations that lead to factors, which further modify chromatin in the vicinity of the DSB and cancer1,2. The generation of DSB is associated with structural propagate the DNA damage response. Given the extensive changes in chro- changes in chromatin and the activation of the protein kinase matin structure associated with the DSB response, it could be expected ataxia-telangiectasia mutated (ATM), a key regulator of the that architectural chromatin-binding proteins, such as the high mobility signalling network of the cellular response to DSB3,4. The group (HMG) proteins, would be involved in this process10–12. interrelationship between DSB-induced changes in chromatin HMG is a superfamily of nuclear proteins that bind to chromatin architecture and the activation of ATM is unclear4. Here we show without any obvious specificity for a particular DNA sequence and affect that the nucleosome-binding protein HMGN1 modulates the the structure and activity of the chromatin fibre10,12,13. We have previ- interaction of ATM with chromatin both before and after DSB ously reported that HMGN1, an HMG protein that binds specifically to formation, thereby optimizing its activation. -
The Mitochondrial Kinase PINK1 in Diabetic Kidney Disease
International Journal of Molecular Sciences Review The Mitochondrial Kinase PINK1 in Diabetic Kidney Disease Chunling Huang * , Ji Bian , Qinghua Cao, Xin-Ming Chen and Carol A. Pollock * Kolling Institute, Sydney Medical School, Royal North Shore Hospital, University of Sydney, St. Leonards, NSW 2065, Australia; [email protected] (J.B.); [email protected] (Q.C.); [email protected] (X.-M.C.) * Correspondence: [email protected] (C.H.); [email protected] (C.A.P.); Tel.: +61-2-9926-4784 (C.H.); +61-2-9926-4652 (C.A.P.) Abstract: Mitochondria are critical organelles that play a key role in cellular metabolism, survival, and homeostasis. Mitochondrial dysfunction has been implicated in the pathogenesis of diabetic kidney disease. The function of mitochondria is critically regulated by several mitochondrial protein kinases, including the phosphatase and tensin homolog (PTEN)-induced kinase 1 (PINK1). The focus of PINK1 research has been centered on neuronal diseases. Recent studies have revealed a close link between PINK1 and many other diseases including kidney diseases. This review will provide a concise summary of PINK1 and its regulation of mitochondrial function in health and disease. The physiological role of PINK1 in the major cells involved in diabetic kidney disease including proximal tubular cells and podocytes will also be summarized. Collectively, these studies suggested that targeting PINK1 may offer a promising alternative for the treatment of diabetic kidney disease. Keywords: PINK1; diabetic kidney disease; mitochondria; mitochondria quality control; mitophagy Citation: Huang, C.; Bian, J.; Cao, Q.; 1. Introduction Chen, X.-M.; Pollock, C.A. -
The C9orf72-Interacting Protein Smcr8 Is a Negative Regulator of Autoimmunity and Lysosomal Exocytosis
Downloaded from genesdev.cshlp.org on October 5, 2021 - Published by Cold Spring Harbor Laboratory Press The C9orf72-interacting protein Smcr8 is a negative regulator of autoimmunity and lysosomal exocytosis Yingying Zhang,1,2,3 Aaron Burberry,1,2,3 Jin-Yuan Wang,1,2,3 Jackson Sandoe,1,2,3 Sulagna Ghosh,1,2,3 Namrata D. Udeshi,4 Tanya Svinkina,4 Daniel A. Mordes,1,2,3,5 Joanie Mok,1,2,3 Maura Charlton,1,2,3 Quan-Zhen Li,6,7 Steven A. Carr,4 and Kevin Eggan1,2,3 1Department of Stem Cell and Regenerative Biology, 2Department of Molecular and Cellular Biology, Harvard University, Cambridge, Massachusetts 02138, USA; 3Stanley Center for Psychiatric Research, Broad Institute of Massachusetts Institute of Technology and Harvard, Cambridge, Massachusetts 02142, USA; 4Proteomics Platform, Broad Institute of MIT and Harvard, Cambridge, Massachusetts 02142, USA; 5Department of Pathology, Massachusetts General Hospital, Boston, Massachusetts 02114, USA; 6Department of Immunology, 7Department of Internal Medicine, University of Texas Southwestern Medical Center, Dallas, Texas 75390, USA While a mutation in C9ORF72 is the most common genetic contributor to amyotrophic lateral sclerosis (ALS), much remains to be learned concerning the function of the protein normally encoded at this locus. To elaborate further on functions for C9ORF72, we used quantitative mass spectrometry-based proteomics to identify interacting proteins in motor neurons and found that its long isoform complexes with and stabilizes SMCR8, which further enables interaction with WDR41. To study the organismal and cellular functions for this tripartite complex, we generated Smcr8 loss-of-function mutant mice and found that they developed phenotypes also observed in C9orf72 loss-of- function animals, including autoimmunity. -
Molecular Profile of Tumor-Specific CD8+ T Cell Hypofunction in a Transplantable Murine Cancer Model
Downloaded from http://www.jimmunol.org/ by guest on September 25, 2021 T + is online at: average * The Journal of Immunology , 34 of which you can access for free at: 2016; 197:1477-1488; Prepublished online 1 July from submission to initial decision 4 weeks from acceptance to publication 2016; doi: 10.4049/jimmunol.1600589 http://www.jimmunol.org/content/197/4/1477 Molecular Profile of Tumor-Specific CD8 Cell Hypofunction in a Transplantable Murine Cancer Model Katherine A. Waugh, Sonia M. Leach, Brandon L. Moore, Tullia C. Bruno, Jonathan D. Buhrman and Jill E. Slansky J Immunol cites 95 articles Submit online. Every submission reviewed by practicing scientists ? is published twice each month by Receive free email-alerts when new articles cite this article. Sign up at: http://jimmunol.org/alerts http://jimmunol.org/subscription Submit copyright permission requests at: http://www.aai.org/About/Publications/JI/copyright.html http://www.jimmunol.org/content/suppl/2016/07/01/jimmunol.160058 9.DCSupplemental This article http://www.jimmunol.org/content/197/4/1477.full#ref-list-1 Information about subscribing to The JI No Triage! Fast Publication! Rapid Reviews! 30 days* Why • • • Material References Permissions Email Alerts Subscription Supplementary The Journal of Immunology The American Association of Immunologists, Inc., 1451 Rockville Pike, Suite 650, Rockville, MD 20852 Copyright © 2016 by The American Association of Immunologists, Inc. All rights reserved. Print ISSN: 0022-1767 Online ISSN: 1550-6606. This information is current as of September 25, 2021. The Journal of Immunology Molecular Profile of Tumor-Specific CD8+ T Cell Hypofunction in a Transplantable Murine Cancer Model Katherine A. -
Retinoic Acid Enhances the Expression of Interferon-Induced Proteins: Evidence for Multiple Mechanisms of Action
Oncogene (1997) 15, 2349 ± 2359 1997 Stockton Press All rights reserved 0950 ± 9232/97 $12.00 Retinoic acid enhances the expression of interferon-induced proteins: evidence for multiple mechanisms of action Luis Pelicano1, Fengsheng Li2, Christian Schindler2 and Mounira K Chelbi-Alix1 1CNRS-UPR 9051; 1, avenue Claude Vellefaux, HoÃpital St Louis, 75010 Paris, France; 2Division of Molecular Medicine, College of Physicians and Surgeons of Columbia University, New York, New York 10032, USA Retinoic acid (RA) and interferons (IFNs) are negative Tyk2, phosphorylate Stat1, Stat2 and Stat3. The IFN- regulators of cell proliferation. In vitro and in vivo, their stimulated gene factor 3 (ISGF3) is formed between combination leads to a more potent growth inhibition. Stat1 and Stat2 (Stat1 : 2) in association with the DNA- However, the molecular mechanisms by which RA and binding protein, p48, a member of the interferon IFNs potentiate each other are not fully understood. As regulatory factor (IRF) family (Fu et al., 1992; some IFN-induced gene products regulate cell growth Schindler et al., 1992). This complex binds to the and/or antiviral activity, we analysed the eects of RA IFN-stimulated response element (ISRE) found in on their expressions. RA increases the level of promoters of IFNa/b-stimulated genes. In addition, 2'5'oligoadenylate synthetase, p68 kinase, the promyelo- homo- and heterodimers of Stat1 and Stat3 (Stat1 : 1, cytic leukemia protein (PML) and Sp100 in both HL-60 Stat3 : 3 and Stat1 : 3) bind to a palindromic version of and WISH cells. Moreover, RA and IFN act coopera- the IFNg-activated site (GAS), regulating the expres- tively to increase the expression of these proteins. -
Antibody-Based Delivery of Cytokine Payloads to Carbonic Anhydrase IX
Published OnlineFirst June 18, 2019; DOI: 10.1158/1535-7163.MCT-18-1301 Large Molecule Therapeutics Molecular Cancer Therapeutics Antibody-Based Delivery of Cytokine Payloads to Carbonic Anhydrase IX Leads to Cancer Cures in Immunocompetent Tumor-Bearing Mice Barbara Ziffels1, Marco Stringhini1, Philipp Probst1, Tim Fugmann2, Theo Sturm2, and Dario Neri1 Abstract Antibody–cytokine fusion proteins can have the potential TNF, IL2, or IL12 as payloads cured all mice in their therapy to increase the density and activity of subsets of leukocytes groups, whereas only a subset of mice was cured by the within the tumor mass. Here, we describe the design, produc- antibody-based delivery of IFNa2. Although the antibody tion, and characterization of four novel antibody–cytokine fusion with TNF mediated a rapid hemorrhagic necrosis of fusion proteins directed against human carbonic anhydrase IX, the tumor mass, a slower regression of the neoplastic lesions a highly validated marker of hypoxia that is overexpressed in (which continued after the last injection) was observed with clear cell renal cell carcinoma and other malignancies. As the other fusion proteins, and treated mice acquired protective immunomodulatory payloads we used TNF, IL2, IFNa2 (cor- anticancer immunity. A high proportion of tumor-infiltrating þ responding to products that are in clinical use), and IL12 (as CD8 T cells was specific to the retroviral antigen AH1; this cytokine potently activates T cells and NK cells). Therapy however, the LGPGREYRAL peptide derived from human experiments were performed in BALB/c mice, bearing CT26 carbonic anhydrase IX was also present on tumor cells. The tumors transfected with human carbonic anhydrase IX, in results described herein provide a rationale for the clinical use order to assess the performance of the fusion proteins in an of fully human antibody–cytokine fusions specific to carbonic immunocompetent setting. -
Mechanism of Action Through an IFN Type I-Independent Responses To
Downloaded from http://www.jimmunol.org/ by guest on September 25, 2021 is online at: average * The Journal of Immunology , 12 of which you can access for free at: 2012; 188:3088-3098; Prepublished online 20 from submission to initial decision 4 weeks from acceptance to publication February 2012; doi: 10.4049/jimmunol.1101764 http://www.jimmunol.org/content/188/7/3088 MF59 and Pam3CSK4 Boost Adaptive Responses to Influenza Subunit Vaccine through an IFN Type I-Independent Mechanism of Action Elena Caproni, Elaine Tritto, Mario Cortese, Alessandro Muzzi, Flaviana Mosca, Elisabetta Monaci, Barbara Baudner, Anja Seubert and Ennio De Gregorio J Immunol cites 33 articles Submit online. Every submission reviewed by practicing scientists ? is published twice each month by Submit copyright permission requests at: http://www.aai.org/About/Publications/JI/copyright.html Receive free email-alerts when new articles cite this article. Sign up at: http://jimmunol.org/alerts http://jimmunol.org/subscription http://www.jimmunol.org/content/suppl/2012/02/21/jimmunol.110176 4.DC1 This article http://www.jimmunol.org/content/188/7/3088.full#ref-list-1 Information about subscribing to The JI No Triage! Fast Publication! Rapid Reviews! 30 days* Why • • • Material References Permissions Email Alerts Subscription Supplementary The Journal of Immunology The American Association of Immunologists, Inc., 1451 Rockville Pike, Suite 650, Rockville, MD 20852 Copyright © 2012 by The American Association of Immunologists, Inc. All rights reserved. Print ISSN: 0022-1767 -
A Computational Approach for Defining a Signature of Β-Cell Golgi Stress in Diabetes Mellitus
Page 1 of 781 Diabetes A Computational Approach for Defining a Signature of β-Cell Golgi Stress in Diabetes Mellitus Robert N. Bone1,6,7, Olufunmilola Oyebamiji2, Sayali Talware2, Sharmila Selvaraj2, Preethi Krishnan3,6, Farooq Syed1,6,7, Huanmei Wu2, Carmella Evans-Molina 1,3,4,5,6,7,8* Departments of 1Pediatrics, 3Medicine, 4Anatomy, Cell Biology & Physiology, 5Biochemistry & Molecular Biology, the 6Center for Diabetes & Metabolic Diseases, and the 7Herman B. Wells Center for Pediatric Research, Indiana University School of Medicine, Indianapolis, IN 46202; 2Department of BioHealth Informatics, Indiana University-Purdue University Indianapolis, Indianapolis, IN, 46202; 8Roudebush VA Medical Center, Indianapolis, IN 46202. *Corresponding Author(s): Carmella Evans-Molina, MD, PhD ([email protected]) Indiana University School of Medicine, 635 Barnhill Drive, MS 2031A, Indianapolis, IN 46202, Telephone: (317) 274-4145, Fax (317) 274-4107 Running Title: Golgi Stress Response in Diabetes Word Count: 4358 Number of Figures: 6 Keywords: Golgi apparatus stress, Islets, β cell, Type 1 diabetes, Type 2 diabetes 1 Diabetes Publish Ahead of Print, published online August 20, 2020 Diabetes Page 2 of 781 ABSTRACT The Golgi apparatus (GA) is an important site of insulin processing and granule maturation, but whether GA organelle dysfunction and GA stress are present in the diabetic β-cell has not been tested. We utilized an informatics-based approach to develop a transcriptional signature of β-cell GA stress using existing RNA sequencing and microarray datasets generated using human islets from donors with diabetes and islets where type 1(T1D) and type 2 diabetes (T2D) had been modeled ex vivo. To narrow our results to GA-specific genes, we applied a filter set of 1,030 genes accepted as GA associated. -
Targeting TSLP-Induced Tyrosine Kinase Signaling Pathways in CRLF2-Rearranged Ph-Like ALL Keith C.S
Published OnlineFirst August 14, 2020; DOI: 10.1158/1541-7786.MCR-19-1098 MOLECULAR CANCER RESEARCH | CANCER GENES AND NETWORKS Targeting TSLP-Induced Tyrosine Kinase Signaling Pathways in CRLF2-Rearranged Ph-like ALL Keith C.S. Sia1, Ling Zhong2, Chelsea Mayoh1, Murray D. Norris1,3, Michelle Haber1, Glenn M. Marshall1,4, Mark J. Raftery2, and Richard B. Lock1,3 ABSTRACT ◥ Philadelphia (Ph)-like acute lymphoblastic leukemia (ALL) is combination cytotoxicity assays using the tyrosine kinase inhi- characterized by aberrant activation of signaling pathways and bitors BMS-754807 and ponatinib that target IGF1R and FGFR1, high risk of relapse. Approximately 50% of Ph-like ALL cases respectively, revealed strong synergy against both cell line and overexpress cytokine receptor-like factor 2 (CRLF2) associated patient-derived xenograft (PDX) models of CRLF2r Ph-like ALL. with gene rearrangement. Activated by its ligand thymic stromal Further analyses also indicated off-target effects of ponatinib in lymphopoietin (TSLP), CRLF2 signaling is critical for the devel- the synergy, and novel association of the Ras-associated protein- opment, proliferation, and survival of normal lymphocytes. To 1 (Rap1) signaling pathway with TSLP signaling in CRLF2r Ph- examine activation of tyrosine kinases regulated by TSLP/CRLF2, like ALL. When tested in vivo, the BMS-754807/ponatinib com- phosphotyrosine (P-Tyr) profiling coupled with stable isotope bination exerted minimal efficacy against 2 Ph-like ALL PDXs, labeling of amino acids in cell culture (SILAC) was conducted associated with low achievable plasma drug concentrations. using two CRLF2-rearranged (CRLF2r) Ph-like ALL cell lines Although this study identified potential new targets in CRLF2r stimulated with TSLP. -
Dux4r, Znf384r and PAX5-P80R Mutated B-Cell Precursor Acute
Acute Lymphoblastic Leukemia SUPPLEMENTARY APPENDIX DUX4r , ZNF384r and PAX5 -P80R mutated B-cell precursor acute lymphoblastic leukemia frequently undergo monocytic switch Michaela Novakova, 1,2,3* Marketa Zaliova, 1,2,3* Karel Fiser, 1,2* Barbora Vakrmanova, 1,2 Lucie Slamova, 1,2,3 Alena Musilova, 1,2 Monika Brüggemann, 4 Matthias Ritgen, 4 Eva Fronkova, 1,2,3 Tomas Kalina, 1,2,3 Jan Stary, 2,3 Lucie Winkowska, 1,2 Peter Svec, 5 Alexandra Kolenova, 5 Jan Stuchly, 1,2 Jan Zuna, 1,2,3 Jan Trka, 1,2,3 Ondrej Hrusak 1,2,3# and Ester Mejstrikova 1,2,3# 1CLIP - Childhood Leukemia Investigation Praguerague, Czech Republic; 2Department of Paediatric Hematology and Oncology, Second Faculty of Medicine, Charles University, Prague, Czech Republic; 3University Hospital Motol, Prague, Czech Republic; 4Department of In - ternal Medicine II, University Hospital Schleswig-Holstein, Kiel, Germany and 5Comenius University, National Institute of Children’s Dis - eases, Bratislava, Slovakia *MN, MZ and KF contributed equally as co-first authors. #OH and EM contributed equally as co-senior authors. ©2021 Ferrata Storti Foundation. This is an open-access paper. doi:10.3324/haematol. 2020.250423 Received: February 18, 2020. Accepted: June 25, 2020. Pre-published: July 9, 2020. Correspondence: ESTER MEJSTRIKOVA - [email protected] Table S1. S1a. List of antibodies used for diagnostic immunophenotyping. Antibody Fluorochrome Clone Catalogue number Manufacturer CD2 PE 39C1.5 A07744 Beckman Coulter CD3 FITC UCHT1 1F-202-T100 Exbio CD4 PE-Cy7 -
HMGB1 in Health and Disease R
Donald and Barbara Zucker School of Medicine Journal Articles Academic Works 2014 HMGB1 in health and disease R. Kang R. C. Chen Q. H. Zhang W. Hou S. Wu See next page for additional authors Follow this and additional works at: https://academicworks.medicine.hofstra.edu/articles Part of the Emergency Medicine Commons Recommended Citation Kang R, Chen R, Zhang Q, Hou W, Wu S, Fan X, Yan Z, Sun X, Wang H, Tang D, . HMGB1 in health and disease. 2014 Jan 01; 40():Article 533 [ p.]. Available from: https://academicworks.medicine.hofstra.edu/articles/533. Free full text article. This Article is brought to you for free and open access by Donald and Barbara Zucker School of Medicine Academic Works. It has been accepted for inclusion in Journal Articles by an authorized administrator of Donald and Barbara Zucker School of Medicine Academic Works. Authors R. Kang, R. C. Chen, Q. H. Zhang, W. Hou, S. Wu, X. G. Fan, Z. W. Yan, X. F. Sun, H. C. Wang, D. L. Tang, and +8 additional authors This article is available at Donald and Barbara Zucker School of Medicine Academic Works: https://academicworks.medicine.hofstra.edu/articles/533 NIH Public Access Author Manuscript Mol Aspects Med. Author manuscript; available in PMC 2015 December 01. NIH-PA Author ManuscriptPublished NIH-PA Author Manuscript in final edited NIH-PA Author Manuscript form as: Mol Aspects Med. 2014 December ; 0: 1–116. doi:10.1016/j.mam.2014.05.001. HMGB1 in Health and Disease Rui Kang1,*, Ruochan Chen1, Qiuhong Zhang1, Wen Hou1, Sha Wu1, Lizhi Cao2, Jin Huang3, Yan Yu2, Xue-gong Fan4, Zhengwen Yan1,5, Xiaofang Sun6, Haichao Wang7, Qingde Wang1, Allan Tsung1, Timothy R.