Tethered Homing Gene Drives: a New Design for Spatially Restricted
Total Page:16
File Type:pdf, Size:1020Kb
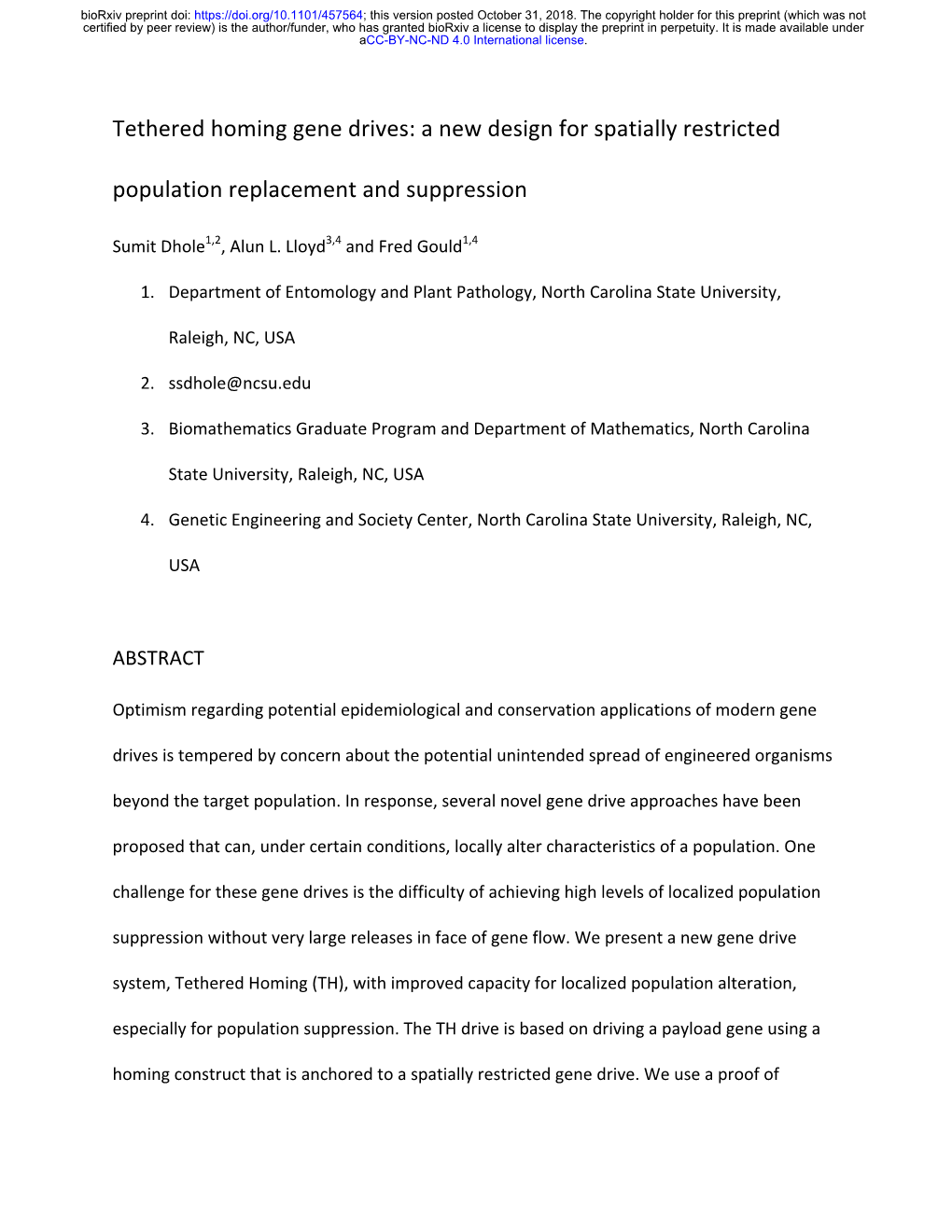
Load more
Recommended publications
-
Estimating Mutation Load in Human Genomes
HHS Public Access Author manuscript Author ManuscriptAuthor Manuscript Author Nat Rev Manuscript Author Genet. Author manuscript; Manuscript Author available in PMC 2016 July 25. Published in final edited form as: Nat Rev Genet. 2015 June ; 16(6): 333–343. doi:10.1038/nrg3931. Estimating Mutation Load in Human Genomes Brenna M. Henn1, Laura R. Botigué1, Carlos D. Bustamante2, Andrew G. Clark3, and Simon Gravel4 1Stony Brook University, SUNY, Department of Ecology and Evolution, 650 Life Sciences Building, Stony Brook, NY, 11794-5245 2Stanford University School of Medicine, Department of Genetics, 291 Campus Drive, Stanford, CA, 94305 3Cornell University, Department of Molecular Biology and Genetics, 526 Campus Road, Ithaca, NY, 14853-2703 4McGill University, Department of Human Genetics and Genome Quebec Innovation Centre, 740 Dr. Penfield Ave, Montreal, QC, H3A 0G1, Canada Abstract Next-generation sequencing technology has facilitated the discovery of millions of variants in human genomes. A sizeable fraction of these alleles are thought to be deleterious. We review the pattern of deleterious alleles as ascertained in genomic data and ask whether human populations differ in their predicted burden of deleterious alleles, a phenomenon known as “mutation load.” We discuss three demographic models that are predicted to affect mutation load and relate these models to the evidence (or the lack thereof) for variation in the efficacy of purifying selection in diverse human genomes. We also discuss why accurate estimation of mutation load depends on assumptions regarding the distribution of dominance and selection coefficients, quantities that are poorly characterized for current genomic datasets. Introduction The process of mutation constantly creates deleterious variation in a population. -
Epistasis and the Mutation Load: a Measurement-Theoretical Approach
Copyright 2001 by the Genetics Society of America Epistasis and the Mutation Load: A Measurement-Theoretical Approach Thomas F. Hansen and GuÈnter P. Wagner Department of Ecology and Evolutionary Biology, Yale University, New Haven, Connecticut 06520-8106 Manuscript received September 9, 2000 Accepted for publication February 15, 2001 ABSTRACT An approximate solution for the mean ®tness in mutation-selection balance with arbitrary order of epistatic interaction is derived. The solution is based on the assumptions of coupling equilibrium and that the interaction effects are multilinear. We ®nd that the effect of m-order epistatic interactions (i.e., interactions among groups of m loci) on the load is dependent on the total genomic mutation rate, U, to the mth power. Thus, higher-order gene interactions are potentially important if U is large and the interaction density among loci is not too low. The solution suggests that synergistic epistasis will decrease the mutation load and that variation in epistatic effects will elevate the load. Both of these results, however, are strictly true only if they refer to epistatic interaction strengths measured in the optimal genotype. If gene interactions are measured at mutation-selection equilibrium, only synergistic interactions among even numbers of genes will reduce the load. Odd-ordered synergistic interactions will then elevate the load. There is no systematic relationship between variation in epistasis and load at equilibrium. We argue that empirical estimates of gene interaction must pay attention to the genetic background in which the effects are measured and that it may be advantageous to refer to average interaction intensities as measured in mutation-selection equilibrium. -
THE MUTATION LOAD in SMALL POPULATIONS HE Mutation Load
THE MUTATION LOAD IN SMALL POPULATIONS MOT00 KIMURAZ, TAKE0 MARUYAMA, and JAMES F. CROW University of Wisconsin, Madison, Wisconsin Received April 29, 1963 HE mutation load has been defined as the proportion by which the population fitness, or any other attribute of interest, is altered by recurrent mutation (MORTON,CROW, and MULLER1956; CROW1958). HALDANE(1937) and MULLER(1950) had earlier shown that this load is largely independent of the harmfulness of the mutant. As long as the selective disadvantage of the mutant is of a larger order of magnitude than the mutation rate and the heterozygote fitness is not out of the range of that of the homozygotes, the load (measured in terms of fitness) is equal to the mutation rate for a recessive mutant and approxi- mately twice the mutation rate for a dominant mutant. A detailed calculation of the value for various degrees of dominance has been given by KIMURA(1 961 ) . In all these studies it has been assumed that the population is so large and the conditions so stable that the frequency of a mutant gene is exactly determined by the mutation rates, dominance, and selection coefficients, with no random fluctuation. However, actual populations are finite and also there are departures from equilibrium conditions because of variations in the various determining factors. Our purpose is to investigate the effect of random drift caused by a finite population number. It would be expected that the load would increase in a small population because the gene frequencies would drift away from the equilibrium values. This was confirmed by our mathematical investigations, but two somewhat unexpected results emerged. -
A Threefold Genetic Allee Effect: Population Size Affects Cross-Compatibility
Genetics: Published Articles Ahead of Print, published on February 3, 2005 as 10.1534/genetics.104.034553 A Threefold Genetic Allee Effect: Population Size Affects Cross-Compatibility, Inbreeding Depression, and Drift Load in the Self-Incompatible Ranunculus reptans Yvonne Willi,* Josh Van Buskirk,† Markus Fischer* ,1 * Institute of Environmental Sciences, University of Zürich, 8057 Zürich, Switzerland † Zoology Department, Melbourne University, Melbourne, VIC 3010, Australia 1 Institute of Biochemistry and Biology, University of Potsdam, 14471 Potsdam, Germany Y. Willi et al., page 2 Running head: INBREEDING DEPRESSION AND DRIFT LOAD Key words: Inbreeding load, fixed drift load, polyploidy, SI system, small population Corresponding author: Yvonne Willi Institut für Umweltwissenschaften Universität Zürich Winterthurerstrasse 190 CH-8057 Zürich, Switzerland telephone: ++ 41-1-635-4067 fax: ++ 41-1-635-5711 email: [email protected] Y. Willi et al. , page 3 ABSTRACT A decline in population size can lead to the loss of allelic variation, increased inbreeding, and the accumulation of genetic load through drift. We estimated the fitness consequences of these processes in offspring of controlled within-population crosses from 13 populations of the self-incompatible, clonal plant Ranunculus reptans. We used allozyme allelic richness as a proxy for long-term population size, which was positively correlated with current population size. Crosses between plants of smaller populations were less likely to be compatible. Inbreeding load, assessed as the slope of the relationship between offspring performance and parental kinship coefficients, was not related to population size, suggesting that deleterious mutations had not been purged from small populations. Offspring from smaller populations were on average more inbred, so inbreeding depression in clonal fitness was higher in small populations. -
Genetic Load in Sexual and Asexual Diploids: Segregation, Dominance and Genetic Drift
Copyright Ó 2007 by the Genetics Society of America DOI: 10.1534/genetics.107.073080 Genetic Load in Sexual and Asexual Diploids: Segregation, Dominance and Genetic Drift Christoph R. Haag1 and Denis Roze2 University of Edinburgh, Institute of Evolutionary Biology, Edinburgh EH9 3JT, United Kingdom Manuscript received March 8, 2007 Accepted for publication April 19, 2007 ABSTRACT In diploid organisms, sexual reproduction rearranges allelic combinations between loci (recombina- tion) as well as within loci (segregation). Several studies have analyzed the effect of segregation on the genetic load due to recurrent deleterious mutations, but considered infinite populations, thus neglecting the effects of genetic drift. Here, we use single-locus models to explore the combined effects of segrega- tion, selection, and drift. We find that, for partly recessive deleterious alleles, segregation affects both the deterministic component of the change in allele frequencies and the stochastic component due to drift. As a result, we find that the mutation load may be far greater in asexuals than in sexuals in finite and/or subdivided populations. In finite populations, this effect arises primarily because, in the absence of segregation, heterozygotes may reach high frequencies due to drift, while homozygotes are still efficiently selected against; this is not possible with segregation, as matings between heterozygotes constantly prod- uce new homozygotes. If deleterious alleles are partly, but not fully recessive, this causes an excess load in asexuals at intermediate population sizes. In subdivided populations without extinction, drift mostly occurs locally, which reduces the efficiency of selection in both sexuals and asexuals, but does not lead to global fixation. -
The Inflated Significance of Neutral Genetic Diversity in Conservation Genetics PERSPECTIVE Jo~Ao C
PERSPECTIVE The inflated significance of neutral genetic diversity in conservation genetics PERSPECTIVE Jo~ao C. Teixeiraa,b,1 and Christian D. Hubera,1 Edited by Andrew G. Clark, Cornell University, Ithaca, NY, and approved December 30, 2020 (received for review July 22, 2020) The current rate of species extinction is rapidly approaching unprecedented highs, and life on Earth presently faces a sixth mass extinction event driven by anthropogenic activity, climate change, and ecological collapse. The field of conservation genetics aims at preserving species by using their levels of genetic diversity, usually measured as neutral genome-wide diversity, as a barometer for evaluating pop- ulation health and extinction risk. A fundamental assumption is that higher levels of genetic diversity lead to an increase in fitness and long-term survival of a species. Here, we argue against the perceived impor- tance of neutral genetic diversity for the conservation of wild populations and species. We demonstrate that no simple general relationship exists between neutral genetic diversity and the risk of species extinc- tion. Instead, a better understanding of the properties of functional genetic diversity, demographic his- tory, and ecological relationships is necessary for developing and implementing effective conservation genetic strategies. conservation genetics | adaptive potential | inbreeding depression | genetic load | species extinction Are Species with Little Genetic Diversity Moreover, low genetic diversity is related to reduced Endangered? individual life span and health, along with a depleted Climate change caused by human activity is currently capacity for population growth (9). In contrast, high responsible for widespread ecological disruption and levels of genetic diversity are often seen as key to habitat destruction, with an ensuing unprecedented promoting population survival and guaranteeing the rate of species loss known as the Anthropocene Mass adaptive potential of natural populations in the face of Extinction (1–4). -
Genetic Load and Mortality in Partially Inbred Populations of Swine Barbara Jane Hicks Iowa State University
Iowa State University Capstones, Theses and Retrospective Theses and Dissertations Dissertations 1967 Genetic load and mortality in partially inbred populations of swine Barbara Jane Hicks Iowa State University Follow this and additional works at: https://lib.dr.iastate.edu/rtd Part of the Agriculture Commons, and the Animal Sciences Commons Recommended Citation Hicks, Barbara Jane, "Genetic load and mortality in partially inbred populations of swine " (1967). Retrospective Theses and Dissertations. 3463. https://lib.dr.iastate.edu/rtd/3463 This Dissertation is brought to you for free and open access by the Iowa State University Capstones, Theses and Dissertations at Iowa State University Digital Repository. It has been accepted for inclusion in Retrospective Theses and Dissertations by an authorized administrator of Iowa State University Digital Repository. For more information, please contact [email protected]. This dissertation has been microfilmed exactly as received 6 8-5957 HICKS, Barbara Jane, 1942- GENETIC LOAD AND MORTALITY IN PARTIALLY INBRED POPULATIONS OF SWINE. Iowa State University, Ph.D., 1967 Agriculture, animal culture University Microfilms, Inc., Ann Arbor, Michigan GENETIC LOAD Am MORTALITY IN PARTIALLY INBRED POPULATIONS OF SWINE "by Barbara Jane Hicks A Dissertation Submitted to the Graduate Faculty in Partial Fulfillment of The Requirements for the Degree of DOCTOR OF PHILOSOPHY Major Subject: Animal Breeding Approved: Signature was redacted for privacy. Signature was redacted for privacy. Heafâ of Major Department Signature was redacted for privacy. Iowa State University Of Science and Technology Ames, Iowa 1967 il TABLE OF CONTENTS Page INTRODUCTION 1 REVIEW OF LITERATURE 2 Genetic Load 2 Litter Size and Mortality l6 SOURCE OF DA.TA. -
Genetic Load”: How the Architects of the Modern Synthesis Became Trapped in a Scientific Ideology Alexandra Soulier
“Genetic Load”: How the Architects of the Modern Synthesis Became Trapped in a Scientific Ideology Alexandra Soulier To cite this version: Alexandra Soulier. “Genetic Load”: How the Architects of the Modern Synthesis Became Trapped in a Scientific Ideology. Transversal: International Journal for the Historiography of Science, Graduate Program in History of Federal University of Minas Gerais, 2018, 4 (4), pp.118-139. 10.24117/2526- 2270.2018.i4.11. hal-01818407 HAL Id: hal-01818407 https://hal.archives-ouvertes.fr/hal-01818407 Submitted on 19 Jun 2018 HAL is a multi-disciplinary open access L’archive ouverte pluridisciplinaire HAL, est archive for the deposit and dissemination of sci- destinée au dépôt et à la diffusion de documents entific research documents, whether they are pub- scientifiques de niveau recherche, publiés ou non, lished or not. The documents may come from émanant des établissements d’enseignement et de teaching and research institutions in France or recherche français ou étrangers, des laboratoires abroad, or from public or private research centers. publics ou privés. Transversal: International Journal for the Historiography of Science, 4 (2018) 118-139 ISSN 2526-2270 www.historiographyofscience.org © The Author 2018 – This is an open access article “Genetic Load”: How the Architects of the Modern Synthesis Became Trapped in a Scientific Ideology Alexandra Soulier1 Abstract The term “genetic load” first emerged in a paper written in 1950 by the geneticist H. Muller. It is a mathematical model based on biological, social, political and ethical arguments describ- ing the dramatic accumulation of disadvantageous mutations in human populations that will occur in modern societies if eugenic measures are not taken. -
Genetic Load and Soft Selection in Ferns
Heredity (1982),49 (2), 191—197 0018-067X/82/05100191$02.OO 1982. The Genetical Society of Great Britain GENETICLOAD AND SOFT SELECTION IN FERNS EDWARD J. KLEKOWSKI, JR. Department of Botany, University of Massachusetts, Amherst, Massachusetts 01003, U.S.A. Received15.iii.82 SUMMARY Genetic load data for two homosporous ferns are summarized and the mean frequency of recessive sporophytic lethals per zygote calculated. Fitty-one spore collections of Onocleasensibilishad a mean of O•587 lethals per zygote, and 129 spore collections of Osmunda regalis had a mean of 239 lethals per zygote. Each spore collection represents a meiotic sample from a single individual (genet). Both species form hermaphroditic gametophytes with extensive capacities for simple polyembryony. Simple polyembryony results in a form of soft selection in panmictic populations and thus higher equilibrium frequencies for lethals are possible than in organisms without simple polyembryony. When ferns are inbred, the high load levels are expressed. Load levels in ferns may reflect a balance between soft selection in panmictic populations and hard selection under conditions of inbreeding. The sheltering effect of simple poly- embryony is demonstrated for both mutational and heterotic load. 1. INTRODUCTION IN practically all species of sexually reproducing, out-crossing organisms, a low frequency of extreme deviant phenotypes segregates each generation. This observation and the finding that inbreeding such species usually results in a higher frequency of such deviant phenotypes has led to the view that most individuals are heterozygous for deleterious or lethal factors (Dobzhansky, 1957). The concept of genetic load is derived from such observations (Muller, 1950; Morton, Crow and Muller, 1956). -
Tethered Homing Gene Drives: a New Design for Spatially Restricted
bioRxiv preprint first posted online Oct. 31, 2018; doi: http://dx.doi.org/10.1101/457564. The copyright holder for this preprint (which was not peer-reviewed) is the author/funder, who has granted bioRxiv a license to display the preprint in perpetuity. It is made available under a CC-BY-NC-ND 4.0 International license. Tethered homing gene drives: a new design for spatially restricted population replacement and suppression Sumit Dhole1,2, Alun L. Lloyd3,4 and Fred Gould1,4 1. Department of Entomology and Plant Pathology, North Carolina State University, Raleigh, NC, USA 2. [email protected] 3. Biomathematics Graduate Program and Department of Mathematics, North Carolina State University, Raleigh, NC, USA 4. Genetic Engineering and Society Center, North Carolina State University, Raleigh, NC, USA ABSTRACT Optimism regarding potential epidemiological and conservation applications of modern gene drives is tempered by concern about the potential unintended spread of engineered organisms beyond the target population. In response, several novel gene drive approaches have been proposed that can, under certain conditions, locally alter characteristics of a population. One challenge for these gene drives is the difficulty of achieving high levels of localized population suppression without very large releases in face of gene flow. We present a new gene drive system, Tethered Homing (TH), with improved capacity for localized population alteration, especially for population suppression. The TH drive is based on driving a payload gene using a homing construct that is anchored to a spatially restricted gene drive. We use a proof of bioRxiv preprint first posted online Oct. 31, 2018; doi: http://dx.doi.org/10.1101/457564. -
Inbreeding Depression and Drift Load in Small Populations at Demographic Disequilibrium
ORIGINAL ARTICLE doi:10.1111/evo.13103 Inbreeding depression and drift load in small populations at demographic disequilibrium Rachel B. Spigler,1,2 Konstantinos Theodorou,3 and Shu-Mei Chang4 1Department of Biology, Temple University, 1900 N. 12th Street, Philadelphia, Pennsylvania 19122 2E-mail: [email protected] 3Biodiversity Conservation Laboratory, Department of Environment, University of the Aegean, University Hill 81100, Mytilene, Greece 4Department of Plant Biology, University of Georgia, 2502 Miller Plant Sciences, Athens, Georgia 30602–7271 Received February 20, 2015 Accepted October 13, 2016 Inbreeding depression is a major driver of mating system evolution and has critical implications for population viability. Theoretical and empirical attention has been paid to predicting how inbreeding depression varies with population size. Lower inbreeding depression is predicted in small populations at equilibrium, primarily due to higher inbreeding rates facilitating purging and/or fixation of deleterious alleles (drift load), but predictions at demographic and genetic disequilibrium are less clear. In this study, we experimentally evaluate how lifetime inbreeding depression and drift load, estimated by heterosis, vary with census (Nc) and effective (estimated as genetic diversity, He) population size across six populations of the biennial Sabatia angularis as well as present novel models of inbreeding depression and heterosis under varying demographic scenarios at disequilibrium (fragmentation, bottlenecks, disturbances). Our experimental study reveals high average inbreeding depression and heterosis across populations. Across our small sample, heterosis declined with He, as predicted, whereas inbreeding depression did not vary with He and actually decreased with Nc. Our theoretical results demonstrate that inbreeding depression and heterosis levels can vary widely across populations at disequilibrium despite similar He and highlight that joint demographic and genetic dynamics are key to predicting patterns of genetic load in nonequilibrium systems. -
Mutation Load: the Fitness of Individuals in Populations Where Deleterious Alleles Are Abundant
ES43CH06-Agrawal ARI 1 October 2012 12:1 Mutation Load: The Fitness of Individuals in Populations Where Deleterious Alleles Are Abundant Aneil F. Agrawal1 and Michael C. Whitlock2 1Department of Ecology & Evolutionary Biology, University of Toronto, Toronto, Ontario, Canada M5S 3B2; email: [email protected] 2Department of Zoology, University of British Columbia, Vancouver, British Columbia, Canada V6T 1Z4; email: [email protected] Annu. Rev. Ecol. Evol. Syst. 2012. 43:115–35 Keywords First published online as a Review in Advance on genetic load, evolutionary theory, population ecology August 28, 2012 The Annual Review of Ecology, Evolution, and Abstract Systematics is online at ecolsys.annualreviews.org by University of Toronto on 11/06/12. For personal use only. Many multicellular eukaryotes have reasonably high per-generation muta- This article’s doi: tion rates. Consequently, most populations harbor an abundance of segre- 10.1146/annurev-ecolsys-110411-160257 gating deleterious alleles. These alleles, most of which are of small effect Copyright c 2012 by Annual Reviews. individually, collectively can reduce substantially the fitness of individuals All rights reserved Annu. Rev. Ecol. Evol. Syst. 2012.43:115-135. Downloaded from www.annualreviews.org relative to what it would be otherwise; this is mutation load. Mutation load 1543-592X/12/1201-0115$20.00 can be lessened by any factor that causes more mutations to be removed per selective death, such as inbreeding, synergistic epistasis, population struc- ture, or harsh environments. The ecological effects of load are not clear-cut because some conditions (such as selection early in life, sexual selection, re- productive compensation, and intraspecific competition) reduce the effects of load on population size and persistence, but other conditions (such as in- terspecific competition and load on resource use efficiency) can cause small amounts of load to have strong effects on the population, even extinction.