Progress in Developing Inhibitors of SARS-Cov-2 3C-Like Protease
Total Page:16
File Type:pdf, Size:1020Kb
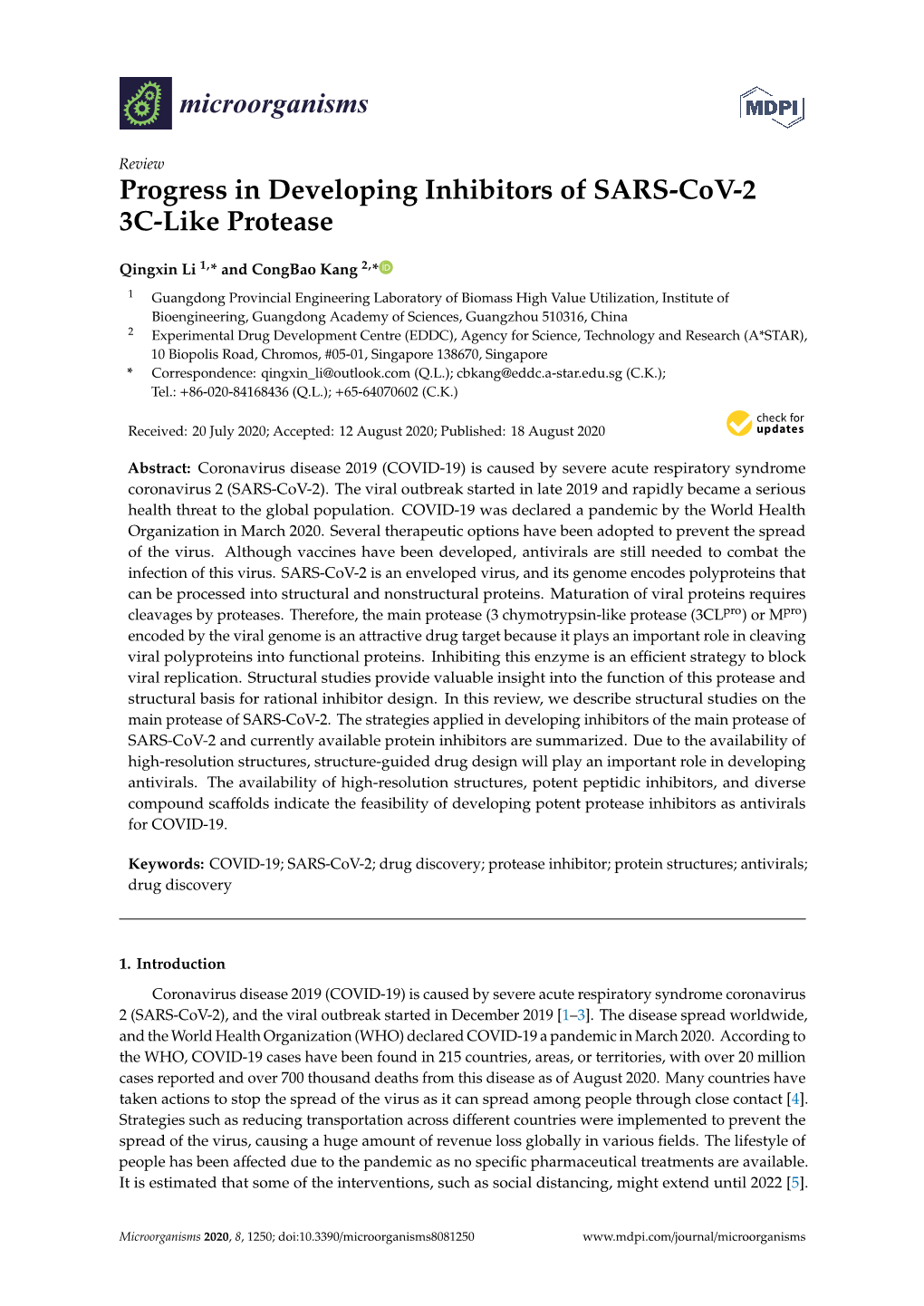
Load more
Recommended publications
-
Enzymes Handling/Processing
Enzymes Handling/Processing 1 Identification of Petitioned Substance 2 3 This Technical Report addresses enzymes used in used in food processing (handling), which are 4 traditionally derived from various biological sources that include microorganisms (i.e., fungi and 5 bacteria), plants, and animals. Approximately 19 enzyme types are used in organic food processing, from 6 at least 72 different sources (e.g., strains of bacteria) (ETA, 2004). In this Technical Report, information is 7 provided about animal, microbial, and plant-derived enzymes generally, and more detailed information 8 is presented for at least one model enzyme in each group. 9 10 Enzymes Derived from Animal Sources: 11 Commonly used animal-derived enzymes include animal lipase, bovine liver catalase, egg white 12 lysozyme, pancreatin, pepsin, rennet, and trypsin. The model enzyme is rennet. Additional details are 13 also provided for egg white lysozyme. 14 15 Chemical Name: Trade Name: 16 Rennet (animal-derived) Rennet 17 18 Other Names: CAS Number: 19 Bovine rennet 9001-98-3 20 Rennin 25 21 Chymosin 26 Other Codes: 22 Prorennin 27 Enzyme Commission number: 3.4.23.4 23 Rennase 28 24 29 30 31 Chemical Name: CAS Number: 32 Peptidoglycan N-acetylmuramoylhydrolase 9001-63-2 33 34 Other Name: Other Codes: 35 Muramidase Enzyme Commission number: 3.2.1.17 36 37 Trade Name: 38 Egg white lysozyme 39 40 Enzymes Derived from Plant Sources: 41 Commonly used plant-derived enzymes include bromelain, papain, chinitase, plant-derived phytases, and 42 ficin. The model enzyme is bromelain. -
Application of Plant Proteolytic Enzymes for Tenderization of Rabbit Meat
Biotechnology in Animal Husbandry 34 (2), p 229-238 , 2018 ISSN 1450-9156 Publisher: Institute for Animal Husbandry, Belgrade-Zemun UDC 637.5.039'637.55'712 https://doi.org/10.2298/BAH1802229D APPLICATION OF PLANT PROTEOLYTIC ENZYMES FOR TENDERIZATION OF RABBIT MEAT Maria Doneva, Iliana Nacheva, Svetla Dyankova, Petya Metodieva, Daniela Miteva Institute of Cryobiology and Food Technology, Cherni Vrah 53, 1407, Sofia, Bulgaria Corresponding author: Maria Doneva, e-mail: [email protected] Original scientific paper Abstract: The purpose of this study is to assess the tenderizing effect of plant proteolytic enzymes upon raw rabbit meat. Tests are performed on rabbit meat samples treated with papain and two vegetal sources of natural proteases (extracts of kiwifruit and ginger root). Two variants of marinade solutions are prepared from each vegetable raw materials– 50% (w/w) and 100 % (w/w), with a duration of processing 2h, 24h, and 48h. Changes in the following physico- chemical characteristics of meat have been observed: pH, water-holding capacity, cooking losses and quantity of free amino acids. Differences in values of these characteristics have been observed, both between control and test samples, as well as depending of treatment duration. For meat samples marinated with papain and ginger extracts, the water-holding capacity reached to 6.74 ± 0.04 % (papain), 5.58 ± 0.09 % (variant 1) and 6.80 ± 0.11 % (variant 2) after 48 hours treatment. In rabbit meat marinated with kiwifruit extracts, a significant increase in WHC was observed at 48 hours, 3.37 ± 0.07 (variant 3) and 6.84 ± 0.11 (variant 4). -
Structure-Based Design of Specific Peptidomimetic Inhibitors of SARS
RSC Advances View Article Online PAPER View Journal | View Issue Antiviral agents against COVID-19: structure-based design of specific peptidomimetic inhibitors of Cite this: RSC Adv., 2020, 10,40244 SARS-CoV-2 main protease Vladimir Frecer *ab and Stanislav Miertusbc Despite the intense development of vaccines and antiviral therapeutics, no specific treatment of coronavirus disease 2019 (COVID-19), caused by the new severe acute respiratory syndrome coronavirus 2 (SARS-CoV-2), is currently available. Recently, X-ray crystallographic structures of a validated pharmacological target of SARS-CoV-2, the main protease (Mpro also called 3CLpro) in complex with peptide-like irreversible inhibitors have been published. We have carried out computer-aided structure- based design and optimization of peptidomimetic irreversible a-ketoamide Mpro inhibitors and their analogues using MM, MD and QM/MM methodology, with the goal to propose lead compounds with improved binding affinity to SARS-CoV-2 Mpro, enhanced specificity for pathogenic coronaviruses, Creative Commons Attribution 3.0 Unported Licence. decreased peptidic character, and favourable drug-like properties. The best inhibitor candidates Received 28th September 2020 designed in this work show largely improved interaction energies towards the Mpro and enhanced Accepted 30th October 2020 specificity due to 6 additional hydrogen bonds to the active site residues. The presented results on new DOI: 10.1039/d0ra08304f SARS-CoV-2 Mpro inhibitors are expected to stimulate further research towards the development of rsc.li/rsc-advances specific anti-COVID-19 drugs. Introduction chymotrypsin-like protease (called main protease Mpro or also 3CLpro), and papain-like protease (PLpro). The 33.8 kDa cysteine pro This article is licensed under a The outbreak of the new severe acute respiratory syndrome protease M (EC 3.4.22.69) encoded in the nsp5 is a key viral coronavirus 2 (SARS-CoV-2) belonging to the b-lineage of the enzyme essential for the viral life cycle of coronaviruses. -
Current IUBMB Recommendations on Enzyme Nomenclature and Kinetics$
Perspectives in Science (2014) 1,74–87 Available online at www.sciencedirect.com www.elsevier.com/locate/pisc REVIEW Current IUBMB recommendations on enzyme nomenclature and kinetics$ Athel Cornish-Bowden CNRS-BIP, 31 chemin Joseph-Aiguier, B.P. 71, 13402 Marseille Cedex 20, France Received 9 July 2013; accepted 6 November 2013; Available online 27 March 2014 KEYWORDS Abstract Enzyme kinetics; The International Union of Biochemistry (IUB, now IUBMB) prepared recommendations for Rate of reaction; describing the kinetic behaviour of enzymes in 1981. Despite the more than 30 years that have Enzyme passed since these have not subsequently been revised, though in various respects they do not nomenclature; adequately cover current needs. The IUBMB is also responsible for recommendations on the Enzyme classification naming and classification of enzymes. In contrast to the case of kinetics, these recommenda- tions are kept continuously up to date. & 2014 The Author. Published by Elsevier GmbH. This is an open access article under the CC BY license (http://creativecommons.org/licenses/by/3.0/). Contents Introduction...................................................................75 Kinetics introduction...........................................................75 Introduction to enzyme nomenclature ................................................76 Basic definitions ................................................................76 Rates of consumption and formation .................................................76 Rate of reaction .............................................................76 -
COVID-19: Living Through Another Pandemic Essam Eldin A
pubs.acs.org/journal/aidcbc Viewpoint COVID-19: Living through Another Pandemic Essam Eldin A. Osman, Peter L. Toogood, and Nouri Neamati* Cite This: https://dx.doi.org/10.1021/acsinfecdis.0c00224 Read Online ACCESS Metrics & More Article Recommendations *sı Supporting Information ABSTRACT: Novel beta-coronavirus SARS-CoV-2 is the pathogenic agent responsible for coronavirus disease-2019 (COVID-19), a globally pandemic infectious disease. Due to its high virulence and the absence of immunity among the general population, SARS- CoV-2 has quickly spread to all countries. This pandemic highlights the urgent unmet need to expand and focus our research tools on what are considered “neglected infectious diseases” and to prepare for future inevitable pandemics. This global emergency has generated unprecedented momentum and scientificefforts around the globe unifying scientists from academia, government and the pharmaceutical industry to accelerate the discovery of vaccines and treatments. Herein, we shed light on the virus structure and life cycle and the potential therapeutic targets in SARS-CoV-2 and briefly refer to both active and passive immunization modalities, drug repurposing focused on speed to market, and novel agents against specific viral targets as therapeutic interventions for COVID-19. s first reported in December 2019, a novel coronavirus, rate of seasonal influenza (flu), which is fatal in only ∼0.1% of A severe acute respiratory syndrome coronavirus 2 (SARS- infected patients.6 In contrast to previous coronavirus CoV-2), caused an outbreak of atypical pneumonia in Wuhan, epidemics (Table S1), COVID-19 is indiscriminately wreaking 1 China, that has since spread globally. The disease caused by havoc globally with no apparent end in sight due to its high this new virus has been named coronavirus disease-2019 virulence and the absence of resistance among the general (COVID-19) and on March 11, 2020 was declared a global population. -
SARS-Cov-2) Papain-Like Proteinase(Plpro
JOURNAL OF VIROLOGY, Oct. 2010, p. 10063–10073 Vol. 84, No. 19 0022-538X/10/$12.00 doi:10.1128/JVI.00898-10 Copyright © 2010, American Society for Microbiology. All Rights Reserved. Papain-Like Protease 1 from Transmissible Gastroenteritis Virus: Crystal Structure and Enzymatic Activity toward Viral and Cellular Substratesᰔ Justyna A. Wojdyla,1† Ioannis Manolaridis,1‡ Puck B. van Kasteren,2 Marjolein Kikkert,2 Eric J. Snijder,2 Alexander E. Gorbalenya,2 and Paul A. Tucker1* EMBL Hamburg Outstation, c/o DESY, Notkestrasse 85, D-22603 Hamburg, Germany,1 and Molecular Virology Laboratory, Department of Medical Microbiology, Center of Infectious Diseases, Leiden University Medical Center, P.O. Box 9600, 2300 RC Leiden, Netherlands2 Received 27 April 2010/Accepted 15 July 2010 Coronaviruses encode two classes of cysteine proteases, which have narrow substrate specificities and either a chymotrypsin- or papain-like fold. These enzymes mediate the processing of the two precursor polyproteins of the viral replicase and are also thought to modulate host cell functions to facilitate infection. The papain-like protease 1 (PL1pro) domain is present in nonstructural protein 3 (nsp3) of alphacoronaviruses and subgroup 2a betacoronaviruses. It participates in the proteolytic processing of the N-terminal region of the replicase polyproteins in a manner that varies among different coronaviruses and remains poorly understood. Here we report the first structural and biochemical characterization of a purified coronavirus PL1pro domain, that of transmissible gastroenteritis virus (TGEV). Its tertiary structure is compared with that of severe acute respiratory syndrome (SARS) coronavirus PL2pro, a downstream paralog that is conserved in the nsp3’s of all coronaviruses. -
DEUBIQUITINATING ACTIVITY of the SARS-Cov PAPAIN-LIKE PROTEASE
DEUBIQUITINATING ACTIVITY OF THE SARS-CoV PAPAIN-LIKE PROTEASE Naina Barretto1, Dalia Jukneliene1, Kiira Ratia2, Zhongbin Chen1,3, Andrew D. Mesecar2 and Susan C. Baker1 1. INTRODUCTION The SARS-CoV replicase polyproteins are processed into 16 nonstructural proteins (nsps) by two viral proteases; a 3C-like protease (3CLpro) and a papain-like protease (PLpro). PLpro processes the amino-terminal end of the replicase polyprotein to release nsp1, nsp2, and nsp3. In this study, we identified a 316 amino acid core catalytic domain for SARS-CoV PLpro that is active in trans-cleavage assays. Interestingly, bioinformatics analysis of the SARS-CoV PLpro domain suggested that this protease may also have deubiquitinating activity because it is predicted to have structural similarity to a cellular deubiquitinase, HAUSP (herpesvirus-associated ubiquitin-specific-protease). Using a purified preparation of the catalytic core domain in an in vitro assay, we demonstrate that PLpro has the ability to cleave ubiquitinated substrates. We also established a FRET- based assay to study the kinetics of proteolysis and deubiquitination by SARS-CoV PLpro. This characterization of a PLpro catalytic core will facilitate structural studies as well as high-throughput assays to identify antiviral compounds. IDENTIFYING A PLpro CATALYTIC CORE DOMAIN Proteolytic processing of the coronavirus replicase polyprotein by 3CLpro and the papain-like proteases is essential for the formation of the mature replicase complex. In light of its role in processing the amino-terminal end of the replicase polyprotein, PLpro is a potential target for the development of antiviral drugs. To identify a catalytically active core domain for PLpro, we performed deletion analysis from the C- and N-terminii of a region of SARS-CoV nsp3, which we had previously shown to be proteolytic active. -
Validation and Invalidation of SARS-Cov-2 Main Protease Inhibitors Using the 2 Flip-GFP and Protease-Glo Luciferase Assays
bioRxiv preprint doi: https://doi.org/10.1101/2021.08.28.458041; this version posted August 30, 2021. The copyright holder for this preprint (which was not certified by peer review) is the author/funder, who has granted bioRxiv a license to display the preprint in perpetuity. It is made available under aCC-BY 4.0 International license. 1 Validation and invalidation of SARS-CoV-2 main protease inhibitors using the 2 Flip-GFP and Protease-Glo luciferase assays 3 Chunlong Ma,a Haozhou Tan,a Juliana Choza,a Yuying Wang,a and Jun Wang,a,* 4 5 6 7 aDepartment of Pharmacology and Toxicology, College of Pharmacy, The University of 8 Arizona, Tucson, USA, 85721. 9 10 *Address correspondence to Jun Wang, [email protected] 11 12 Running title: Validation/invalidation of SARS-CoV-2 Mpro inhibitors 13 14 15 16 17 18 19 20 21 22 1 bioRxiv preprint doi: https://doi.org/10.1101/2021.08.28.458041; this version posted August 30, 2021. The copyright holder for this preprint (which was not certified by peer review) is the author/funder, who has granted bioRxiv a license to display the preprint in perpetuity. It is made available under aCC-BY 4.0 International license. 23 24 25 Graphical abstract 26 27 Flip-GFP and Protease-Glo luciferase assays, coupled with the FRET and thermal shift 28 binding assays, were applied to validate the reported SARS-CoV-2 Mpro inhibitors. 29 30 31 32 33 34 35 36 37 38 39 2 bioRxiv preprint doi: https://doi.org/10.1101/2021.08.28.458041; this version posted August 30, 2021. -
A Rationale for Targeting Sentinel Innate Immune Signaling of Group 1 House Dust Mite Allergens Th
Molecular Pharmacology Fast Forward. Published on July 5, 2018 as DOI: 10.1124/mol.118.112730 This article has not been copyedited and formatted. The final version may differ from this version. MOL #112730 1 Title Page MiniReview for Molecular Pharmacology Allergen Delivery Inhibitors: A Rationale for Targeting Sentinel Innate Immune Signaling of Group 1 House Dust Mite Allergens Through Structure-Based Protease Inhibitor Design Downloaded from molpharm.aspetjournals.org Jihui Zhang, Jie Chen, Gary K Newton, Trevor R Perrior, Clive Robinson at ASPET Journals on September 26, 2021 Institute for Infection and Immunity, St George’s, University of London, Cranmer Terrace, London SW17 0RE, United Kingdom (JZ, JC, CR) State Key Laboratory of Microbial Resources, Institute of Microbiology, Chinese Academy of Sciences, Beijing, P.R. China (JZ) Domainex Ltd, Chesterford Research Park, Little Chesterford, Saffron Walden, CB10 1XL, United Kingdom (GKN, TRP) Molecular Pharmacology Fast Forward. Published on July 5, 2018 as DOI: 10.1124/mol.118.112730 This article has not been copyedited and formatted. The final version may differ from this version. MOL #112730 2 Running Title Page Running Title: Allergen Delivery Inhibitors Correspondence: Professor Clive Robinson, Institute for Infection and Immunity, St George’s, University of London, SW17 0RE, UK [email protected] Downloaded from Number of pages: 68 (including references, tables and figures)(word count = 19,752) 26 (main text)(word count = 10,945) Number of Tables: 3 molpharm.aspetjournals.org -
Serine Proteases from Nematode and Protozoan Parasites
Proc. Natl. Acad. Sci. USA Vol. 86, pp. 4863-4867, July 1989 Biochemistry Serine proteases from nematode and protozoan parasites: Isolation of sequence homologs using generic molecular probes (molecular evolution/trypsin/cysteine/active sites/polymerase chain reaction) JUDY A. SAKANARI*t, CATHERINE E. STAUNTON*t, ANN E. EAKIN§, CHARLES S. CRAIK§, AND JAMES H. MCKERROW* *Department of Pathology and §Department of Pharmaceutical Chemistry, University of California, San Francisco, CA 94143; and tCornell University Medical College, New York, NY 10021 Communicated by Russell F. Doolittle, March 27, 1989 ABSTRACT Serine proteases are one of the biologically represent some of the world's greatest health problems (16- most important and widely distributed families of enzymes. 18). Parasite proteases may facilitate invasion of host tissue, Isolation of serine protease genes from organisms of widely metabolism of host proteins, and evasion of the host immune diverged phylogenetic groups would provide a basis for study- response. A generic molecular technology for isolation of ing their biological function, the relationship between structure serine protease genes from diverse sources would be of and function, and the molecular evolution of these enzymes. immense value in expanding the data base for serine proteases Serine proteases for which little structural information is and to further our understanding of the function of these known are those that are important in the pathogenesis of enzymes in a variety of organisms. parasitic nematode and protozoan diseases. Identification and In the first step of developing such a generic molecular isolation of protease genes from these organisms is a critical strategy, we report the isolation offour serine protease genes first step in understanding their function for the parasite and from a parasitic nematode using degenerate oligonucleotide possibly suggesting innovative approaches to arresting para- primers and genomic DNA. -
High Molecular Weight Kininogen Is an Inhibitor of Platelet Calpain
High molecular weight kininogen is an inhibitor of platelet calpain. A H Schmaier, … , D Schutsky, R W Colman J Clin Invest. 1986;77(5):1565-1573. https://doi.org/10.1172/JCI112472. Research Article Recent studies from our laboratory indicate that a high concentration of platelet-derived calcium-activated cysteine protease (calpain) can cleave high molecular weight kininogen (HMWK). On immunodiffusion and immunoblot, antiserum directed to the heavy chain of HMWK showed immunochemical identity with alpha-cysteine protease inhibitor--a major plasma inhibitor of tissue calpains. Studies were then initiated to determine whether purified or plasma HMWK was also an inhibitor of platelet calpain. Purified alpha-cysteine protease inhibitor, alpha-2-macroglobulin, as well as purified heavy chain of HMWK or HMWK itself inhibited purified platelet calpain. Kinetic analysis revealed that HMWK inhibited platelet calpain noncompetitively (Ki approximately equal to 5 nM). Incubation of platelet calpain with HMWK, alpha-2- macroglobulin, purified heavy chain of HMWK, or purified alpha-cysteine protease inhibitor under similar conditions resulted in an IC50 of 36, 500, 700, and 1,700 nM, respectively. The contribution of these proteins in plasma towards the inhibition of platelet calpain was investigated next. Normal plasma contained a protein that conferred a five to sixfold greater IC50 of purified platelet calpain than plasma deficient in either HMWK or total kininogen. Reconstitution of total kininogen deficient plasma with purified HMWK to normal levels (0.67 microM) completely corrected the subnormal inhibitory activity. However, reconstitution of HMWK deficient plasma to normal levels of low molecular weight kininogen (2.4 microM) did not fully correct the subnormal calpain inhibitory capacity […] Find the latest version: https://jci.me/112472/pdf High Molecular Weight Kininogen Is an Inhibitor of Platelet Calpain Alvin H. -
VEGF-A Induces Angiogenesis by Perturbing the Cathepsin-Cysteine
Published OnlineFirst May 12, 2009; DOI: 10.1158/0008-5472.CAN-08-4539 Published Online First on May 12, 2009 as 10.1158/0008-5472.CAN-08-4539 Research Article VEGF-A Induces Angiogenesis by Perturbing the Cathepsin-Cysteine Protease Inhibitor Balance in Venules, Causing Basement Membrane Degradation and Mother Vessel Formation Sung-Hee Chang,1 Keizo Kanasaki,2 Vasilena Gocheva,4 Galia Blum,5 Jay Harper,3 Marsha A. Moses,3 Shou-Ching Shih,1 Janice A. Nagy,1 Johanna Joyce,4 Matthew Bogyo,5 Raghu Kalluri,2 and Harold F. Dvorak1 Departments of 1Pathology and 2Medicine, and the Center for Vascular Biology Research, Beth Israel Deaconess Medical Center and Harvard Medical School, and 3Departments of Surgery, Children’s Hospital and Harvard Medical School, Boston, Massachusetts; 4Cancer Biology and Genetics Program, Memorial Sloan-Kettering Cancer Center, New York, New York; and 5Department of Pathology, Stanford University, Stanford, California Abstract to form in many transplantable mouse tumor models are mother Tumors initiate angiogenesis primarily by secreting vascular vessels (MV), a blood vessel type that is also common in many endothelial growth factor (VEGF-A164). The first new vessels autochthonous human tumors (2, 3, 6–8). MV are greatly enlarged, to form are greatly enlarged, pericyte-poor sinusoids, called thin-walled, hyperpermeable, pericyte-depleted sinusoids that form mother vessels (MV), that originate from preexisting venules. from preexisting venules. The dramatic enlargement of venules We postulated that the venular enlargement necessary to form leading to MV formation would seem to require proteolytic MV would require a selective degradation of their basement degradation of their basement membranes.