Prompt D Meson Production in Pp, P-Pb and Pb-Pb Collisions at LHC
Total Page:16
File Type:pdf, Size:1020Kb
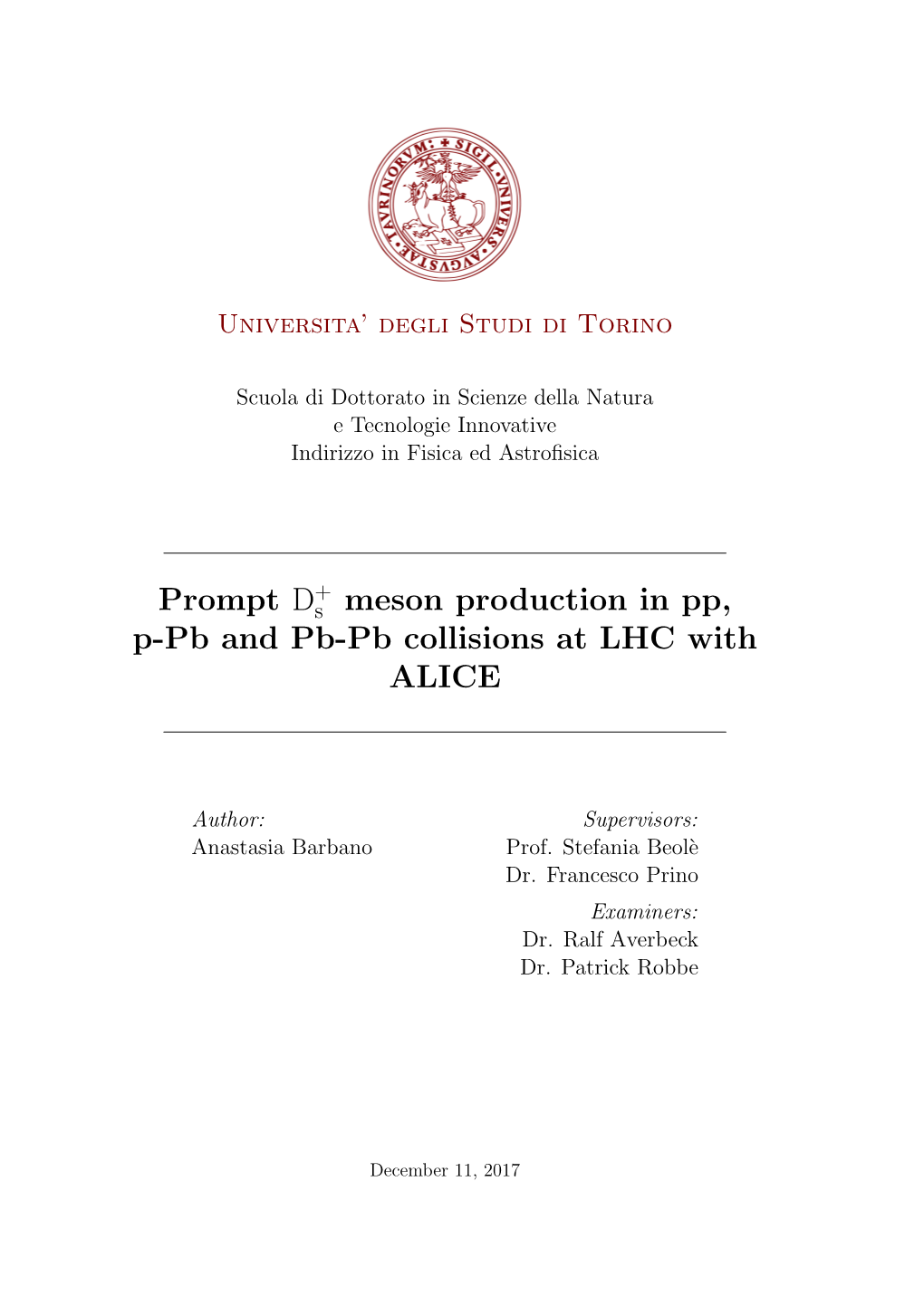
Load more
Recommended publications
-
Strange Hadrocharmonium ∗ M.B
Physics Letters B 798 (2019) 135022 Contents lists available at ScienceDirect Physics Letters B www.elsevier.com/locate/physletb Strange hadrocharmonium ∗ M.B. Voloshin a,b,c, a William I. Fine Theoretical Physics Institute, University of Minnesota, Minneapolis, MN 55455, USA b School of Physics and Astronomy, University of Minnesota, Minneapolis, MN 55455, USA c Institute of Theoretical and Experimental Physics, Moscow, 117218, Russia a r t i c l e i n f o a b s t r a c t Article history: It has been recently suggested that the charged charmoniumlike resonances Zc (4100) and Zc (4200) Received 23 May 2019 are two states of hadrocharmonium, related by the charm quark spin symmetry in the same way Received in revised form 12 August 2019 as the lowest charmonium states ηc and J/ψ. It is pointed out here that in this picture one might Accepted 16 September 2019 expect existence of their somewhat heavier strange counterparts, Zcs, decaying to ηc K and J/ψ K . Some Available online 11 October 2019 expected properties of such charmoniumlike strange resonances are discussed that set benchmarks for Editor: B. Grinstein their search in the decays of the strange Bs mesons. © 2019 The Author. Published by Elsevier B.V. This is an open access article under the CC BY license 3 (http://creativecommons.org/licenses/by/4.0/). Funded by SCOAP . Numerous new resonances recently uncovered near the open down to about 4220 MeV), has been recently invoked [11]for de- charm and open bottom thresholds, the so-called XYZ states, ap- scription of the charged charmoniumlike resonances Zc(4100) and parently do not fit in the standard quark-antiquark template and Zc(4200) observed respectively in the decay channels ηcπ [12] 1 contain light constituents in addition to a heavy quark-antiquark and J/ψπ [13]. -
Minimal Flavour Violation in a Custodial Two Higgs Doublet Model
Universit´ecatholique de Louvain Secteur des sciences et technologies Institut de recherche en math´ematique et physique Centre for Cosmology, Particle Physics and Phenomenology Minimal Flavour Violation in a custodial Two Higgs Doublet Model Doctoral dissertation presented by Elvira Cerver´oGarc´ıa in fulfilment of the requirements for the degree of Doctor in Sciences Jury Prof. Vincent Lemaˆıtre (chairman) UCL, Belgium Prof. Jean-Marc G´erard (advisor) UCL, Belgium Prof. Fabio Maltoni UCL, Belgium Prof. Christophe Delaere UCL, Belgium Prof. Michel Tytgat ULB, Belgium Prof. Christopher Smith Universit´eGrenoble Alpes, France July 2017 ”Everything comes gradually and at its appointed hour” Ovid I would like to thank my advisor, Professor Jean-Marc G´erard, for giving me the opportunity of carrying out and achieving this work. His passion and en- thusiasm have encouraged me to deepen in the understanding of nature through scientific research. I also want to thank all the members of my jury, Professors Vincent Lemaˆıtre, Fabio Maltoni, Christophe Delaere, Michel Tytgat and Christopher Smith. I am honoured that they have taken the time to assess this work and contribute to its improvement with their relevant questions and remarks. My gratitude extends to the numerous professors, fellow PhD students and stu- dents that have in one way or another influenced my learning since I arrived to this univerity already twelve years ago. I am unable to name each and everyone of them, but I can only say thank you for helping me to become a physicist and making me always feel at home. I cannot finish without thanking all the people around me that have brought this project to completion. -
1) Direct Top-Quark Decay Width Measurement in the $T\Bar{T}
Publications of Ian Shipsey (September, 2017) 1) Direct top-quark decay width measurement in the $t\bar{t}$ lepton+jets channel at $\sqrt{s}=8 \rm{TeV}$ with the ATLAS experiment By ATLAS Collaboration (Morad Aaboud et al.). arXiv:1709.04207 [hep-ex]. Submitted to Eur. Phys. J. C. 2) Search for a scalar partner of the top quark in the jets plus missing transverse momentum final state at $\sqrt{s}$=13 TeV with the ATLAS detector By ATLAS Collaboration (Morad Aaboud et al.). arXiv:1709.04183 [hep-ex]. Submitted to JHEP. 3) Measurement of $\tau$ polarisation in $Z/\gamma^{*}\rightarrow\tau\tau$ decays in proton-proton collisions at $\sqrt{s}=8$ TeV with the ATLAS detector By ATLAS Collaboration (Morad Aaboud et al.). arXiv:1709.03490 [hep-ex]. Submitted to Eur. Phys. J. C. 3a) An Electro - Optical Test System for Optimising Operating Conditions of CCD sensors for LSST D.P. Weatherill, R. Plackett, K. Arndt and I.P.J. Shipsey To appear in JINST 2017. 4) Measurement of quarkonium production in proton--lead and proton--proton collisions at $5.02$ $\mathrm{TeV}$ with the ATLAS detector By ATLAS Collaboration (Morad Aaboud et al.). arXiv:1709.03089 [nucl-ex]. Submitted to Eur. Phys. J. C. 4a) Characterisation of the Medipix3 detector for 60 and 80 keV electrons By J.A. Mir et al.. To appear in Ultramicroscopy Vol. 182 November 2017, Pages 44-53 http://www.sciencedirect.com/science/article/pii/S0304399116303989 5) Measurement of longitudinal flow de-correlations in Pb+Pb collisions at $\sqrt{s_\mathrm{NN}} = 2.76$ and 5.02 TeV with the ATLAS detector By ATLAS Collaboration (Morad Aaboud et al.). -
Measurement of B Meson Production Cross-Sections in Proton-Proton
EUROPEAN ORGANIZATION FOR NUCLEAR RESEARCH (CERN) CERN-PH-EP-2013-095 LHCb-PAPER-2013-004 Oct. 1, 2013 Measurement of B meson production cross-sections inp proton-proton collisions at s = 7 TeV The LHCb collaborationy Abstract The production cross-sections of B mesons are measured in pp collisions at a centre-of- mass energy of 7 TeV, using data collected with the LHCb detector corresponding to −1 + 0 0 a integrated luminosity of 0:36 fb . The B , B and Bs mesons are reconstructed + + 0 ∗0 0 in the exclusive decays B ! J= K , B ! J= K and Bs ! J= φ, with J= ! µ+µ−, K∗0 ! K+π− and φ ! K+K−. The differential cross-sections are measured as functions of B meson transverse momentum pT and rapidity y, in the range 0 < pT < 40 GeV=c and 2:0 < y < 4:5. The integrated cross-sections in the same pT and y ranges, including charge-conjugate states, are measured to be σ(pp ! B+ + X) = 38:9 ± 0:3 (stat:) ± 2:5 (syst:) ± 1:3 (norm:) µb; σ(pp ! B0 + X) = 38:1 ± 0:6 (stat:) ± 3:7 (syst:) ± 4:7 (norm:) µb; 0 σ(pp ! Bs + X) = 10:5 ± 0:2 (stat:) ± 0:8 (syst:) ± 1:0 (norm:) µb; arXiv:1306.3663v2 [hep-ex] 1 Oct 2013 where the third uncertainty arises from the pre-existing branching fraction measure- ments. Submitted to JHEP c CERN on behalf of the LHCb collaboration, license CC-BY-3.0. yAuthors are listed on the following pages. ii LHCb collaboration R. -
Heavy Hadrons Spectra on Lattice Using NRQCD
EPJ Web of Conferences 175, 05031 (2018) https://doi.org/10.1051/epjconf/201817505031 Lattice 2017 Heavy hadrons spectra on lattice using NRQCD Protick Mohanta1, and Subhasish Basak1 1National Institute of Science Education and Research Bhubaneswar, P.O. Jatni, Khurda 752050, Odisha, India Abstract. Comparison of radial excitation energies to masses show that the velocity of b quark is very non-relativistic in bottomonium states. In a mixed system like charmed B meson, the b quark has less velocity than it has in bottomonium states and in strange B meson it is even slower. So one can use NRQCD for the b quark in those systems. Using overlap and hisq action for the s and c quarks and NRQCD for b quark we simulated spectra of charmed and strange B mesons and also few other baryons. 1 Introduction Lattice methods are powerful techniques in analyzing the spectrum of hadrons. However for hadrons containing heavy quarks particularly bottom quark are difficult to analyze. For spectrum calculation it is necessary that aM << 1. For light quarks it is true but for charm quark aMc > 0.7 and for bottom quark aMb > 2 with lattice spacing a = 0.12fm. However in hadrons containing heavy quarks the velocities of heavy quarks are non-relativistic. Comparison of masses of heavy quarkonium to the masses of heavy quarks also indicates the same. For example MΥ = 9390 MeV whereas 2 M = 8360 MeV (MS Scheme) and M = 3096 MeV whereas 2 M = 2580 × b J/ψ × c MeV. This is in contrast with mesons and baryons that are made up of light quarks for example mass of pions M 140 MeV whereas masses of up or down quark is few MeV. -
Arxiv:1702.00766V2 [Hep-Ex] 19 Dec 2017
EUROPEAN ORGANIZATION FOR NUCLEAR RESEARCH CERN-PH-EP-2017-020 30 January 2017 Measurement of D-meson production at mid-rapidity in pp collisions p at s = 7 TeV ALICE Collaboration∗ Abstract 0 + ∗+ + The production cross sections for prompt charmed mesons D ,D ,Dp and Ds were measured at mid-rapidity in proton–proton collisions at a centre-of-mass energy s = 7 TeV with the ALICE detector at the Large Hadron Collider (LHC). D mesons were reconstructed from their decays 0 − + + − + + ∗+ 0 + + + − + + D ! K p ,D ! K p p ,D ! D p ,Ds ! fp ! K K p , and their charge conjugates. With respect to previous measurements in the same rapidity region, the coverage in transverse momentum (pT) is extended and the uncertainties are reduced by a factor of about two. The accuracy on the estimated total cc production cross section is likewise improved. The measured pT-differential cross sections are compared with the results of three perturbative QCD calculations. arXiv:1702.00766v2 [hep-ex] 19 Dec 2017 c 2017 CERN for the benefit of the ALICE Collaboration. Reproduction of this article or parts of it is allowed as specified in the CC-BY-4.0 license. ∗See Appendix A for the list of collaboration members p D-meson production in pp collisions at s = 7 TeV ALICE Collaboration 1 Introduction In high-energy hadronic collisions heavy quarks are produced by hard scatterings between partons of the two incoming hadrons. The production cross section of hadrons with charm or beauty quarks is calculated in the framework of Quantum Chromodynamics (QCD) and factorised as a convolution of the hard scattering cross sections at partonic level, the parton distribution functions (PDFs) of the incoming hadrons and the non-perturbative fragmentation functions of heavy quarks to heavy-flavour hadrons. -
Precision Measurements of B0s Mesons in the CMS Experiment At
HIP Internal Report Series HIP-2017-03 B0 Precision measurements of s mesons in the CMS experiment at the LHC Terhi Järvinen Department of Physics Faculty of Science UNIVERSITY OF HELSINKI ACADEMIC DISSERTATION To be presented with the permission of the Faculty of Science of the University of Helsinki, for public criticism in the auditorium E204 at 10 o’clock, on December 18th, 2017. Helsinki 2017 Supervisors Professor Paula Eerola, University of Helsinki Dr. Giacomo Fedi, University of Pisa and INFN Pisa Pre-examiners Reader Lars Eklund, University of Glasgow Dr. Weiming Yao, Lawrence Berkeley National Laboratory Opponent Professor Ulrik Egede, Imperial College London ISSN 1455-0563 ISBN 978-951-51-1271-2 (paperback) Helsinki University Print (Unigrafia Oy) ISBN 978-951-51-1272-9 (pdf) http://ethesis.helsinki.fi Electronic Publications at the University of Helsinki HELSINGIN YLIOPISTO — HELSINGFORS UNIVERSITET — UNIVERSITY OF HELSINKI Tiedekunta — Fakultet — Faculty Laitos — Institution — Department Faculty of Science Department of Physics Tekijä — Författare — Author Terhi Järvinen Työn nimi — Arbetets titel — Title 0 Precision measurements of Bs mesons in the CMS experiment at the LHC Oppiaine — Läroämne — Subject Physics Työn laji — Arbetets art — Level Aika — Datum — Month and year Sivumäärä — Sidoantal — Number of pages PhD Thesis November 2017 181 pages Tiivistelmä — Referat — Abstract 0 Two precision measurements are performed to Bs data. The primary measurement of the thesis 0 is the effective lifetime of the Bs meson decaying in the J/ψφ(1020) state. The effective lifetime analysis uses 2012 data recorded by the Compact Muon Solenoid (CMS) experiment. The data are collected with a center-of-mass energy of 8 TeV and correspond to an integrated luminosity of −1 19.7 fb . -
Abolhassan Jawahery 1
CURRICULUM VITAE– Abolhassan Jawahery Education: Ph.D. Tufts University June 1981 Physics M.S. Tufts University December 1977 Physics B.S. Tehran University, Iran June 1976 Physics Employment Background: Gus T. Zorn Professor, Dept of Physics, Univ. of Maryland, 2005-present Professor, Dept. of Physics, Univ. of Maryland, 1998-2005 Associate Professor, Dept. of Physics, Univ. of Maryland, 1992-1998 Assistant Professor, Dept. of Physics, Univ. of Maryland, 1987-1992 Research Assistant Professor, Syracuse University 1986-1987 Research Associate, Syracuse University 1981-1986 Visiting Scientist Positions Cornell Electron Storage Ring (CESR), Cornell Univ. 1981-1987 CERN Laboratory, Geneva, Switzerland 1990 Stanford Linear Accelerator Center (SLAC) 2001-2002 Stanford Linear Accelerator Center (SLAC) 2006-present Honors and Award Elected Fellow of the American Physical Society (APS) (2004) Distinguished Faculty Graduate Research Board award (2001) UMD Graduate Research Board Semester award (2000) UMD Graduate Research Board Research support award (1988) Professional Activities Postitions in International Research Collaborations Spokesperson, BaBar Experiment, Stanford Linear Accelerator Center (SLAC), 2006-2008. Physics Analysis Coordinator, BaBar experiment, SLAC, 2001-2002. Physics Analysis Coordinator, CLEO experiment, Cornell Electron Storage Ring, 1987-88. Convener of B physics Group, OPAL experiment at CERN, 1990. Editorial positions Associate Editor, Annual Review of Nuclear and Particle Science, 2003-present Member, Particle Data Group (PDG) collaboration, 2002-2005 1 Conference Advisory Boards and Conference Organizations Member, International advisory comm., CKM workshop, Nagoya, Japan, Dec. 12-16, 2006. Convener, Super B Factory Workshop, Hawaii, April 20-22, 2005. Convener, Division of Par- ticles and Fields Symposium, APS meeting in Philadelphia, April 4-8, 2003. -
Mixing Particles Quark Compositions Are Their Own the Junction Could Be Enhanced Antiparticles
The decay products of a pair of neutral B mesons formed in the disintegration of an upsilon (4S) resonance as observed by the ARGUS experiment at the DORIS electron-positron storage ring at the DESY Laboratory in Hamburg. This shows that the neutral B mesons and their antiparticles appear to mix. tures with a velocity of 104 cm/s. At the liquid helium surface, rotons have a 30% probability of evaporating a helium atom, so the problem of detecting one neu trino is transformed into the prob lem of detecting 107 to 108 eva porated helium atoms with bolo meters mounted above the liquid surface. The physics of the elec tron energy loss in liquid helium as well as the reflectivity of rotons from the liquid surface have to be further investigated. Norman Booth and his collabo rators from Cambridge and Queen Mary College have developed a novel indium detector for a solar ï(4S)-^B°B° neutrino experiment, having suc cessfully worked out a method to grow indium crystals over super conducting tunnel junctions. The energy released when a solar neu BO -p^*- + trino is captured in indium is mostly transformed into phonons. These TTl D° »rr D" can break up Cooper pairs in the junction, leading to quasiparticles. >K27T27T2 The tunnel junction acts as a semi permeable membrane letting the quasiparticles pass, thus giving rise to a signal current, albeit very small. However the sensitivity of Mixing particles quark compositions are their own the junction could be enhanced antiparticles. considerably by trapping the qua The ARGUS experiment (a DESY / Other electrically neutral mesons siparticles in another superconduc Dortmund / Heidelberg / IPP Ca are distinguished from their anti tor of lower gap in the region of nada / Kansas / Ljubljana / Lund / particles by a quark label (quantum the tunnel junction. -
Encounters with Strong Fields
switching and space reflection (CP) sitive to background, consisted of mally this (spin-orbit) coupling is become useful, and the neutral reconstructing one neutral B meson very small, about a thousandth of kaons provide a rich scenario for and tagging the second by the an electronvolt at 10tesla the subtleties of the weak force. charge of an accompanying ener (100 kgauss). Last year the UA1 experiment getic lepton. Five mixing candi If a particle is relativistic (travell at CERN's proton-antiproton col dates were found, where only ing with a velocity comparable to lider provided evidence for an ana about one was expected due to that of light), it experiences a highly logous mixing of the neutral background, again a suggestive velocity-dependent field. Thus if B mesons (see October 1986 signal. an electron moves so fast that the issue, page 17). In today's 'Standard Model', six magnetic field it 'sees' reaches Neutral B mesons exist in two varieties of quark are grouped into about 4 x 109tesla, the energy varieties, with and without strange three doublets (up and down, due to spin alignment becomes quarks. The lighter non-strange strange and charm, beauty and comparable to the electron's mass, particles can be isolated by looking top), and all the corresponding and interesting effects become below the threshold for production quantum number changes pro possible. of the strange quark variety. duced by the weak force are des Using external fields, even high The ARGUS experiment studied cribed by the 'Kobayashi-Maska- energy beams from a particle the formation of neutral non- wa' (KM) matrix. -
Measurement of D-Meson Production at Mid-Rapidity in Pp Collisions at √S=7 Tev
Lawrence Berkeley National Laboratory Recent Work Title Measurement of D-meson production at mid-rapidity in pp collisions at √s=7 TeV Permalink https://escholarship.org/uc/item/2rx7h7sb Journal European Physical Journal C, 77(8) ISSN 1434-6044 Authors Acharya, S Adamová, D Aggarwal, MM et al. Publication Date 2017-08-01 DOI 10.1140/epjc/s10052-017-5090-4 Peer reviewed eScholarship.org Powered by the California Digital Library University of California Eur. Phys. J. C (2017) 77:550 DOI 10.1140/epjc/s10052-017-5090-4 Regular Article - Experimental Physics Measurement of D-meson√ production at mid-rapidity in pp collisions at s = 7TeV ALICE Collaboration CERN, 1211 Geneva 23, Switzerland Received: 19 February 2017 / Accepted: 20 July 2017 © CERN for the benefit of the ALICE collaboration 2017. This article is an open access publication Abstract The production cross sections for prompt respectively. Calculations based on kT factorisation exist 0 + ∗+ + α charmed mesons D ,D ,D and Ds were measured at only at leading order (LO) in s [2,8,9]. All these calcu- mid-rapidity√ in proton–proton collisions at a centre-of-mass lations provide a good description of the production cross energy s = 7 TeV with the ALICE detector at the Large sections of D and B mesons in proton–proton (and proton– Hadron Collider (LHC). D mesons were reconstructed from antiproton) collisions at centre-of-mass energies from 0.2 0 − + + − + + ∗+ their decays D → K π ,D → K π π ,D → to 13 TeV over a wide pT range at both central and for- 0π + + → φπ+ → − +π + D ,Ds K K , and their charge conju- ward rapidities (see e.g. -
Measurement of D-Meson Production at Mid-Rapidity in Pp Collisions at Root S=7 Tev
Measurement of D-meson production at mid-rapidity in pp collisions at root s=7 TeV Acharya, S.; Adamova, D.; Aggarwal, M.M.; Rinella, G.A.; Agnello, Maria; Agrawal, N.; Ahammed, Z.; Ahmad, N.; U. Ahn, S.; Aiola, S.; Akindinov, A.; Alam, SN; Albuquerque, DSD; Aleksandrov, D.; Alessandro, B.; Bearden, Ian; Christensen, Christian Holm; Gulbrandsen, Kristjan Herlache; Gaardhøje, Jens Jørgen; Nielsen, Børge Svane; Bilandzic, Ante; Chojnacki, Marek; Zaccolo, Valentina; Zhou, You; Gajdosova, Katarina; Bourjau, Christian Alexander; Pacik, Vojtech; Pimentel, Lais Ozelin de Lima Published in: European Physical Journal C DOI: 10.1140/epjc/s10052-017-5090-4 Publication date: 2017 Document version Publisher's PDF, also known as Version of record Citation for published version (APA): Acharya, S., Adamova, D., Aggarwal, M. M., Rinella, G. A., Agnello, M., Agrawal, N., Ahammed, Z., Ahmad, N., U. Ahn, S., Aiola, S., Akindinov, A., Alam, SN., Albuquerque, DSD., Aleksandrov, D., Alessandro, B., Bearden, I., Christensen, C. H., Gulbrandsen, K. H., Gaardhøje, J. J., ... Pimentel, L. O. D. L. (2017). Measurement of D- meson production at mid-rapidity in pp collisions at root s=7 TeV. European Physical Journal C, (8), [550]. https://doi.org/10.1140/epjc/s10052-017-5090-4 Download date: 27. sep.. 2021 Eur. Phys. J. C (2017) 77:550 DOI 10.1140/epjc/s10052-017-5090-4 Regular Article - Experimental Physics Measurement of D-meson√ production at mid-rapidity in pp collisions at s = 7TeV ALICE Collaboration CERN, 1211 Geneva 23, Switzerland Received: 19 February 2017 / Accepted: 20 July 2017 © CERN for the benefit of the ALICE collaboration 2017.