UNDERSTANDING the KINETICS and DYNAMICS of RADIATION-INDUCED REACTION PATHWAYS in CARBON MONOXIDE ICE at 10 K Corey S
Total Page:16
File Type:pdf, Size:1020Kb
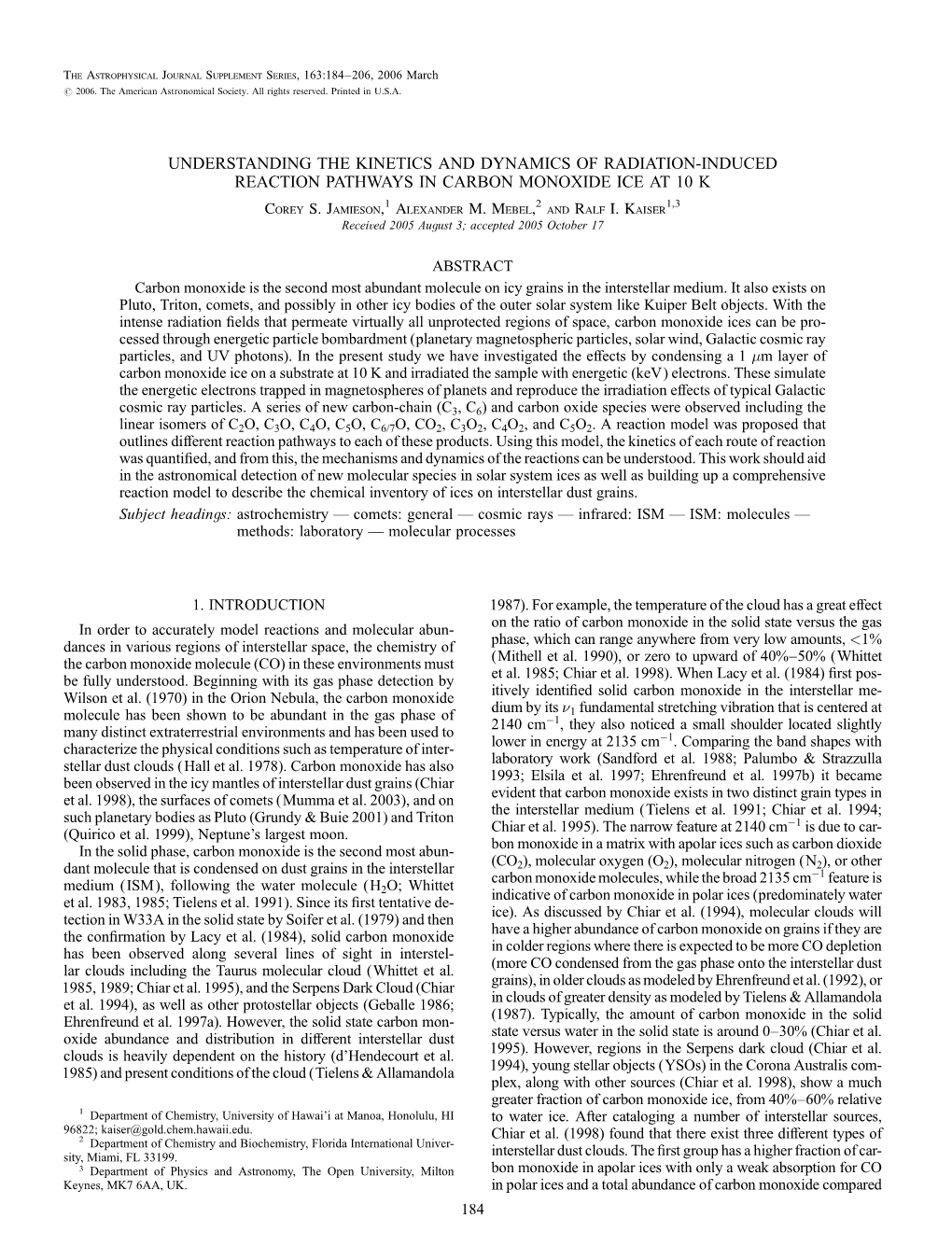
Load more
Recommended publications
-
Some Unusual, Astronomically Significant Organic Molecules
'lL-o Thesis titled: Some Unusual, Astronomically Significant Organic Molecules submitted for the Degree of Doctor of Philosophy (Ph,D.) by Salvatore Peppe B.Sc. (Hons.) of the Department of Ghemistty THE UNIVERSITY OF ADELAIDE AUSTRALIA CRUC E June2002 Preface Gontents Contents Abstract IV Statement of Originality V Acknowledgments vi List of Figures..... ix 1 I. Introduction 1 A. Space: An Imperfect Vacuum 1 B. Stellff Evolution, Mass Outflow and Synthesis of Molecules 5 C. Astronomical Detection of Molecules......... l D. Gas Phase Chemistry.. 9 E. Generation and Detection of Heterocumulenes in the Laboratory 13 L.2 Gas Phase Generation and Characterisation of Ions.....................................16 I. Gas Phase Generation of Ions. I6 A. Positive Ions .. I6 B. Even Electron Negative Ions 17 C. Radical Anions 2t tr. Mass Spectrometry 24 A. The VG ZAB 2}lF Mass Spectrometer 24 B. Mass-Analysed Ion Kinetic Energy Spectrometry......... 25 III. Characterisation of Ions.......... 26 A. CollisionalActivation 26 B. Charge Reversal.... 28 C. Neutralisation - Reionisation . 29 D. Neutral Reactivity. JJ rv. Fragmentation Behaviour ....... 35 A. NegativeIons.......... 35 Preface il B. Charge Inverted Ions 3l 1.3 Theoretical Methods for the Determination of Molecular Geometries and Energetics..... ....o........................................ .....39 L Molecular Orbital Theory........ 39 A. The Schrödinger Equation.... 39 B. Hartree-Fock Theory ..44 C. Electron Correlation ..46 D. Basis sets............ .51 IL Transition State Theory of Unimolecular Reactions ......... ................... 54 2. Covalently Bound Complexes of CO and COz ....... .........................58 L Introduction 58 tr. Results and Discussion........... 59 Part A: Covalently bound COz dimers (OzC-COr)? ............ 59 A. Generation of CzO¿ Anions 6I B. NeutralCzO+........ -
CURRICULUM VITAE Name: William Michael Irvine Born: August 31, 1936; Los Angeles, California, USA Educational Background: 1957
CURRICULUM VITAE Name: William Michael Irvine Born: August 31, 1936; Los Angeles, California, USA Educational Background: 1957 Pomona College B.A. (summa cum laude) 1959 Harvard University M.A. (Physics) 1961 Harvard University Ph.D. (Physics) Professional Employment: 1961-62 Leiden University, Holland NATO Post-doc. Fellow 1962-66 Harvard Coll./Smith. Ast. Obs. Research Fellow/Physicist 1964-66 Harvard University Lecturer 1966-68 University of Massachusetts Associate Professor 1966-79 University of Massachusetts Chair, Astronomy Program 1966-78 Five College Astronomy Dept. Chairman 1969-2006 University of Massachusetts Professor 1973-74 Onsala Space Obs. (Sweden) Visiting Professor 1977 (summer) Kanazawa Inst. Tech. (Japan) Visiting Professor 1979-81 Onsala Space Obs. (Sweden) Acting Scientific Director 1985-1995 Five College Radio Astron. Obs. Director 1990 (winter) National Astron. Obs. (Japan) Visiting Professor 2004 (fall) INAOE (Mexico) Visiting Scientist 2006-present University of Massachusetts Professor Emeritus Research Specialization: Interstellar Medium, Planetary Science, Astrobiology Selected Scholarly Activities: COSPAR (Commissions F, B.1) American Astronomical Society (Heinemann Prize Committee, 1994-97; Nominating Committee, 1989-92, Chairman, 1990-91; Division for Planetary Sciences, Chairman, 1973-74, DPS Committee, 1972-75, 1978-81). Harlow Shapley Visiting Lecturer, AAS, 1975-76, 1978-79. American Geophysical Union. Royal Astronomical Society International Astronomical Union (Commissions 15, 16, 34, 51: Vice-President 2006- 2009; President 2009- 2012; Past President 2012-2015; Division F: Executive Committee 2012- 2015). International Scientific Radio Union. 1 International Society for the Study of the Origin of Life (Councillor, 2008-2011). Committee on Astmospheric Radiative Transfer, Radiation Commiss., IAMAP, 1973-81. Icarus Editorial Board, 1975-76, Associate Editor, 1976-91. -
CHEMISTRY Catholic Junior College
1 Catholic Junior College JC 2 Preliminary Examinations Higher 2 CANDIDATE NAME CLASS 2T CHEMISTRY 9729/01 Paper 1 Multiple Choice Tuesday 3 September 2019 1 hour Additional Materials: Multiple Choice Answer Sheet Data Booklet READ THESE INSTRUCTIONS FIRST Write in soft pencil. Do not use staples, paper clips, glue or correction fluid. Write your name, class and NRIC/FIN number on the Answer Sheet in the spaces provided. There are thirty questions on this paper. Answer all questions. For each question there are four possible answers A, B, C and D. Choose the one you consider correct and record your choice in soft pencil on the separate Answer Sheet. Read the instructions on the Answer Sheet very carefully. Each correct answer will score one mark. A mark will not be deducted for a wrong answer. Any rough working should be done in this booklet. The use of an approved scientific calculator is expected, where appropriate. This document consists of 15 printed pages and 1 blank page. [Turn over 2 3 −3 1 In a titration experiment, 15 cm of 0.10 mol dm phosphorous acid, H3PO3 was found to react with 10 cm3 of 0.30 mol dm−3 sodium hydroxide, NaOH. Using this information, deduce the formula of the salt formed in this neutralisation process. A NaH2PO3 C Na3PO3 B Na2HPO3 D Na3PO4 2 50 cm3 of a mixture of ethene and propane gas were exploded in excess oxygen. The volume of the residual gases was found to be 200 cm3. On being shaken with potassium hydroxide, the final volume was found to be 90 cm3. -
Discovery of HC3O in Space: the Chemistry of O-Bearing Species in TMC-1? J
Astronomy & Astrophysics manuscript no. 39351_final ©ESO 2020 October 12, 2020 Letter to the Editor + Discovery of HC3O in space: The chemistry of O-bearing species in TMC-1? J. Cernicharo1, N. Marcelino1, M. Agúndez1, Y. Endo2, C. Cabezas1, C. Bermúdez1, B. Tercero3; 4, and P. de Vicente4 1 Grupo de Astrofísica Molecular, Instituto de Física Fundamental (IFF-CSIC), C/ Serrano 121, 28006 Madrid, Spain. e-mail: : [email protected] 2 Department of Applied Chemistry, Science Building II, National Chiao Tung University, 1001 Ta-Hsueh Rd., Hsinchu 30010, Taiwan 3 Observatorio Astronómico Nacional (IGN), C/ Alfonso XII, 3, 28014, Madrid, Spain. 4 Centro de Desarrollos Tecnológicos, Observatorio de Yebes (IGN), 19141 Yebes, Guadalajara, Spain. Received; accepted ABSTRACT Using the Yebes 40m and IRAM 30m radio telescopes, we detected a series of harmonically related lines with a rotational constant B0=4460.590±0.001 MHz and a distortion constant D0=0.511±0.005 kHz towards the cold dense core TMC-1. High-level-of-theory + ab initio calculations indicate that the best possible candidate is protonated tricarbon monoxide, HC3O . We have succeeded in producing this species in the laboratory and observed its Ju-Jl = 2-1 and 3-2 rotational transitions. Hence, we report the discovery + of HC3O in space based on our observations, theoretical calculations, and laboratory experiments. We derive an abundance ratio + N(C3O)/N(HC3O )∼7. The high abundance of the protonated form of C3O is due to the high proton affinity of the neutral species. The chemistry of O-bearing species is modelled, and predictions are compared to the derived abundances from our data for the most prominent O-bearing species in TMC-1. -
River Valley High School Year 6 Preliminary Examination Ii
RIVER VALLEY HIGH SCHOOL YEAR 6 PRELIMINARY EXAMINATION II CANDIDATE NAME CLASS 6 CENTRE INDEX 0 0 NUMBER S 3 0 4 4 NUMBER H2 CHEMISTRY 9729/01 Paper 1 Multiple Choice 21 Sep 2017 1 hour Additional Materials: Multiple Choice Answer Sheet Data Booklet READ THESE INSTRUCTIONS FIRST Write in soft pencil. Do not use staples, paper clips, highlighters, glue or correction fluid. Write your name, class and index number on the Optical Answer Sheet in the spaces provided. There are thirty questions on this paper. Answer all questions. For each question there are four possible answers A, B, C and D. Choose the one you consider correct and record your choice in soft pencil on the Optical Answer Sheet. Read the instructions on the Answer Sheet very carefully. Each correct answer will score one mark. A mark will not be deducted for a wrong answer. Any rough working should be done in this booklet. The use of an approved scientific calculator is expected, where appropriate. _____________________________________________________________________________ This document consists of 16 printed pages and 0 blank pages. River Valley High School 9729/01 [Turn over 2017 Preliminary Examinations II KiasuExamPaper.com 2 For each question there are four possible answers, A, B, C and D. Choose the one you consider to be correct. 1 Use of the Data Booklet is relevant to this question. At room temperature and pressure, a sample of 2 dm3 of polluted air was passed through limewater so that all the carbon dioxide present was precipitated as calcium carbonate. The mass of calcium carbonate formed was 0.05 g. -
River Valley High School Year 6 Preliminary Examination Ii
RIVER VALLEY HIGH SCHOOL YEAR 6 PRELIMINARY EXAMINATION II CANDIDATE NAME CLASS 6 CENTRE INDEX 0 0 NUMBER S 3 0 4 4 NUMBER H1 CHEMISTRY 8872/01 Paper 1 Multiple Choice 21 Sep 2017 50 mins Additional Materials: Multiple Choice Answer Sheet Data Booklet READ THESE INSTRUCTIONS FIRST Write in soft pencil. Do not use staples, paper clips, highlighters, glue or correction fluid. Write your name, class and index number on the Optical Answer Sheet in the spaces provided. There are thirty questions on this paper. Answer all questions. For each question there are four possible answers A, B, C and D. Choose the one you consider correct and record your choice in soft pencil on the Optical Answer Sheet. Read the instructions on the Answer Sheet very carefully. Each correct answer will score one mark. A mark will not be deducted for a wrong answer. Any rough working should be done in this booklet. The use of an approved scientific calculator is expected, where appropriate. _____________________________________________________________________________ This document consists of 14 printed pages. River Valley High School 8872/01 [Turn over 2017 Preliminary Examinations II 2 Section A For each question there are four possible answers, A, B, C and D. Choose the one you consider to be correct. 1 Use of the Data Booklet is relevant to this question. At room temperature and pressure, a sample of 2 dm3 of polluted air was passed through limewater so that all the carbon dioxide present was precipitated as calcium carbonate. The mass of calcium carbonate formed was 0.05 g. -
ETUDE EN ONDES MILLIMETRIQUES ET SUBMILLIMETRIQUES D'especes REACTIVES : Détection D'ions Et De Radicaux Libres Au Laboratoire Et Dans Le Milieu Interstellaire
laboratoire de spectroscopie hertzienne Sc, 5:; ;., 1 No d'ordre : 684 présentée à L'UNIVERSITE DES SCIENCES ET TECHNIQUES DE LlLLE FLANDRES ARTOIS Par Claire DEMUYNCK ETUDE EN ONDES MILLIMETRIQUES ET SUBMILLIMETRIQUES D'ESPECES REACTIVES : Détection d'ions et de radicaux libres au laboratoire et dans le milieu interstellaire Soutenue le 30 Mai 1986 devant la Commission d'Examen Membres du Jury : Président : J. LEQUEUX, Astronome titulaire - Observatoire de MARSEILLE Rapporteurs : J.L. DESTOMBES, Chargé de Recherches - Université de LlLLE I E. HI ROTA, Professeur - lnstitute for Molecular Science - OKAZAKI (Japon) A. OMONT, Professeur - Université de GRENOBLE M. WINNEWISSER, Professeur - Université de GIESSEN (R.F.A.) Examinateurs : G. JOURNEL, Professeur - Université de LlLLE 1 S. LEACH, Directeur de Recherches - Université de PARIS XI B. MACKE, Professeur - Université de LILLE I R. WERTHEIMER, Professeur Emerite - Université de LlLLE 1 UNIVERSITE DES SCIENCES ET TECHNIQUES DE LlLLE FLANDRES ARTOIS - U.F.R. DE PHYSIQUE REMERCIEMENTS Ce ;t(tav&, quX. a &té aé&é à L'UnhvmiAé de LXe 7, dam Le 1abotra;tahe de Spe&oacopie ff &Zienne (U. A. 244), n'a pu &the mené à bien que ghiice au concorn de diadérted dac/tewcn davohabL~n : L' é;trtoLte cofibona;tion qui a ' uk Gdabfie avec La as.ttophyaicie~ a &té un Uément ;tauAl à 6aLt d&cinid powz Le développanent de noa ;frtavaux, & C' ut Jamu LEQUEUX qui, avec 1' en;thous&me &t géného- aaé qu' on -eLu con&, uk à L' otLigine de noa conta& avec lu comu- muté decl /radiaa;ttLanom~n~/ta.nçain. Qu'il lfitouve id L' exphennion de ma hecunMainaance, non aeuLemen;t pou avoh accepXE k phenidence de ce jmy, mdin auilai pom Ra con~iancee.t L'amLZLé qu'a noun a Roujo~ kémoigné~cl, & pou Le aou;tien commqutd a appotclté à no&e équipe e-t à L' euemble du Labom,to&e. -
Recent Observations of Organic Molecules in Nearby Cold, Dark Interstellar Clouds
RECENT OBSERVATIONS OF ORGANIC MOLECULES IN NEARBY COLD, DARK INTERSTELLAR CLOUDS H. Suzuki, M. Ohishi, M. Morimoto, and N. Kaifu Nobeyama Radio Observatory Nobeyama, Minamisaku, Nagano 38413, Japan P. Friberg and W.M. Irvine Department of Physics and Astronomy, and Five College Radio Astronomy Observatory University of Massachusetts, Amherst, MA 01003 H. E. Matthews Herzberg Institute for Astrophysics National Research Council of Canada Ottawa, Ontario, K1A 0R6, Canada S. Saito Institute for Molecular Science Okazaki 444, Japan ABSTRACT. We report recent investigations of the organic chemistry of relatively nearby cold, dark interstellar clouds. Specifically, we confirm the presence of interstellar tricarbon monoxide (C3O) in Taurus Molecular Cloud 1 (TMC-1); report the first detection in such regions of acetaldehyde (CH3CHO), the most complex oxygen-containing organic molecule yet found in dark clouds; report the first astronomical detec tion of several molecular rotational transitions, including the J=18-17 and 14-13 transitions of cyanodiacetylene (HC5N), the loi~Ooo tran sition of acetaldehyde, and the J=5-4 transition of C3O; and set a significant upper limit on the abundance of cyanocarbene (HCCN) as a result of the first reported interstellar search for this molecule. 1. INTRODUCTION Throughout most of the history of spectral line radio astronomy those concentrations of the interstellar medium thought to exhibit the most complex chemistry have been the giant molecular clouds, including par ticularly the region Sgr B2 located near the center of the Milky Way Galaxy and the Orion molecular cloud, the closest such region to the solar system. Such giant molecular clouds are the most massive objects in our Galaxy and are obvious sites of current massive star formation. -
Subject Index
Cambridge University Press 978-1-107-16928-9 — Molecular Astrophysics A. G. G. M. Tielens Index More Information Subject Index ab-initio quantum chemistry, 50 anorthite, 487, 488 absorption spectroscopy, 37–41 apolar species, 33 accretion disk, 452 appearance energy, 293, 299, 302 accretion rate, 226 arenes, 33 acetylenic species, 331, 351, 402–404, 412, 512 aromatic hydrocarbons, 5, 7, 33 acid, 30, 35, 397 Aromatic Infrared Band, 258, 568–576, 592 actanoids, 23 Aromatic Infrared Band, assignments, 578 action integral, 229, 230 Aromatic Infrared Band, plateau emission, 573, 574 activity, non-ideal behavior, 142, 147 Aromatic Infrared Band, profiles, 569–572, adduct formation, 304, 312 575, 581 AGB, 402, 542, 549, 604–609 Aromatic Infrared Band, spectral fits, 575, 576 AGN toroid, 11 Aromatic Infrared Band, strength variation, AIB, see Aromatic Infrared Band 572, 573 albite, 487, 488 aromatic molecules, 397 alcohols, 7, 31, 34, 35, 397, 398 aromaticity, 260–263 aldehydes, 7, 31, 34, 35, 397, 398, 479, 508 Arrhenius law, 291 aliphatic group, 580, 584 astrobiology, 2, 4 alkali halides, 219 asymmetric top, 60, 64, 84, 118 alkali metals, 23 atomic force microscope (AFM), 46, 51 alkaline, 30 atomic number, 21, 23–25 alkaline earth metals, 23 aufbau principle, 22, 29 alkanes, 31, 32, 397, 402 autoionization, 193, 198, 269, 300 alkenes, 31, 32, 183, 261, 397, 402 alkynes, 31, 32, 183, 397, 402 B3LYP functional, 52 aluminum oxide, 145, 146, 148, 487, 488 backward reaction, 142 ambipolar diffusion, 383, 392, 394, 407, 455 bandhead, 83 ambipolar diffusion -
Arxiv:1409.6565V1 [Astro-Ph.SR] 23 Sep 2014 Bevtroato´Mc Ainl(A,IN.Clealfons Calle IGN)
The origin of complex organic molecules in prestellar cores C. Vastel1,2 Universit´ede Toulouse, UPS-OMP, IRAP, Toulouse, France CNRS, IRAP, 9 Av. colonel Roche, BP 44346, 31028 Toulouse Cedex 4, France [email protected] C. Ceccarelli3,4 and B. Lefloch3,4 Univ. Grenoble Alpes, IPAG, F-38000 Grenoble, France CNRS, IPAG, F-38000 Grenoble, France R. Bachiller4 Observatorio Astron´omico Nacional (OAN, IGN). Calle Alfonso XII,3. 28014 Madrid, Spain Received - ; accepted - ABSTRACT Complex organic molecules (COMs) have been detected in a variety of environments, including cold prestellar cores. Given the low temperature of these objects, these last detections challenge existing models. We report here new observations towards the prestellar core L1544. They are based on an unbiased spectral survey of the 3mm band at the IRAM-30m telescope, as part of the Large Program ASAI. The observations allow us to provide the full census of the oxygen bearing COMs in this source. We detected tricarbon monoxide, methanol, acetaldehyde, formic acid, ketene, and propyne with abundances varying from 5 × 10−11 to 6 × 10−9. The non-LTE analysis of the methanol lines shows that they are likely emitted at the border of the core, at a radius 4 −3 of ∼ 8000 AU where T ∼ 10 K and nH2 ∼ 2× 10 cm . Previous works have shown arXiv:1409.6565v1 [astro-ph.SR] 23 Sep 2014 that water vapour is enhanced in the same region because of the photodesorption of water ices. We propose that a non-thermal desorption mechanism is also responsible for the observed emission of methanol and COMs from the same layer. -
The Chemistry of O-Bearing Species in TMC-1? J
A&A 642, L17 (2020) Astronomy https://doi.org/10.1051/0004-6361/202039351 & c ESO 2020 Astrophysics LETTER TO THE EDITOR + Discovery of HC3O in space: The chemistry of O-bearing species in TMC-1? J. Cernicharo1, N. Marcelino1, M. Agúndez1, Y. Endo2, C. Cabezas1, C. Bermúdez1, B. Tercero3,4, and P. de Vicente4 1 Grupo de Astrofísica Molecular, Instituto de Física Fundamental (IFF-CSIC), C/ Serrano 121, 28006 Madrid, Spain e-mail: [email protected] 2 Department of Applied Chemistry, Science Building II, National Chiao Tung University, 1001 Ta-Hsueh Rd., Hsinchu 30010, Taiwan 3 Observatorio Astronómico Nacional (IGN), C/ Alfonso XII, 3, 28014 Madrid, Spain 4 Centro de Desarrollos Tecnológicos, Observatorio de Yebes (IGN), 19141 Yebes, Guadalajara, Spain Received 6 September 2020 / Accepted 30 September 2020 ABSTRACT Using the Yebes 40m and IRAM 30m radio telescopes, we detected a series of harmonically related lines with a rotational constant B0 = 4460.590 ± 0.001 MHz and a distortion constant D0 = 0.511 ± 0.005 kHz towards the cold dense core TMC-1. High-level-of- + theory ab initio calculations indicate that the best possible candidate is protonated tricarbon monoxide, HC3O . We have succeeded in producing this species in the laboratory and observed its Ju − Jl = 2–1 and 3–2 rotational transitions. Hence, we report the discovery + of HC3O in space based on our observations, theoretical calculations, and laboratory experiments. We derive an abundance ratio + N(C3O)/N(HC3O ) ∼ 7. The high abundance of the protonated form of C3O is due to the high proton affinity of the neutral species. -
No.1-84 March
Monash • eVI8W VVhot's New InEducation,Research and Community Service Registered lly Australia Posl- publication No. VBG 2204 1-84 The English language is a problem lor a great many migrants. according to a recent survey by the Monash Centre for Migrant Studies. Language train ing in the workplace and in work hours needs ISSN0159-950 to be promoted more effectively, the study says. Photo : Herve Alleaume. Survey reveals migrant language problems A SURVEY conducted by the Monash Housebound women. young unemployed the length of residence in Australia and the Centre for Migrant Studies has revealed adults, factory workers {especially those in ability to speak and understand English, widespread problems in speaking. jobs vulnerable to the pressure of structural Forty-eight per cent of those under 30 had understanding. reading end writing and technological change} and the aged were a lower than minimum "survival" level of English among overseas-born adults Jiving chosen as special target groups, proficiency in speaking. In writing. the figure in Melbourne's western suburbs. The migrants were interviewed and given was 70 per cent. Older people appeared to The study. conducted by Mr John McKay. the Australian Second Language' Proficiency have more problems with reading and writing Ms Susan Manton and Assoclete Profes Rating {ASLPRI test to test their proficiency English than with understanding it. sor Michael Clyne for the Department of in English, Different language groups differed also in Immigration and Ethnic Affa irs. has been The tests showed that in English speaking their ability to use the English language . publ ished in the Adult Migrant Education skills.