Evaluating Genetic Diversity in the Critically Endangered Orange-Bellied Parrot: Informing Species Management
Total Page:16
File Type:pdf, Size:1020Kb
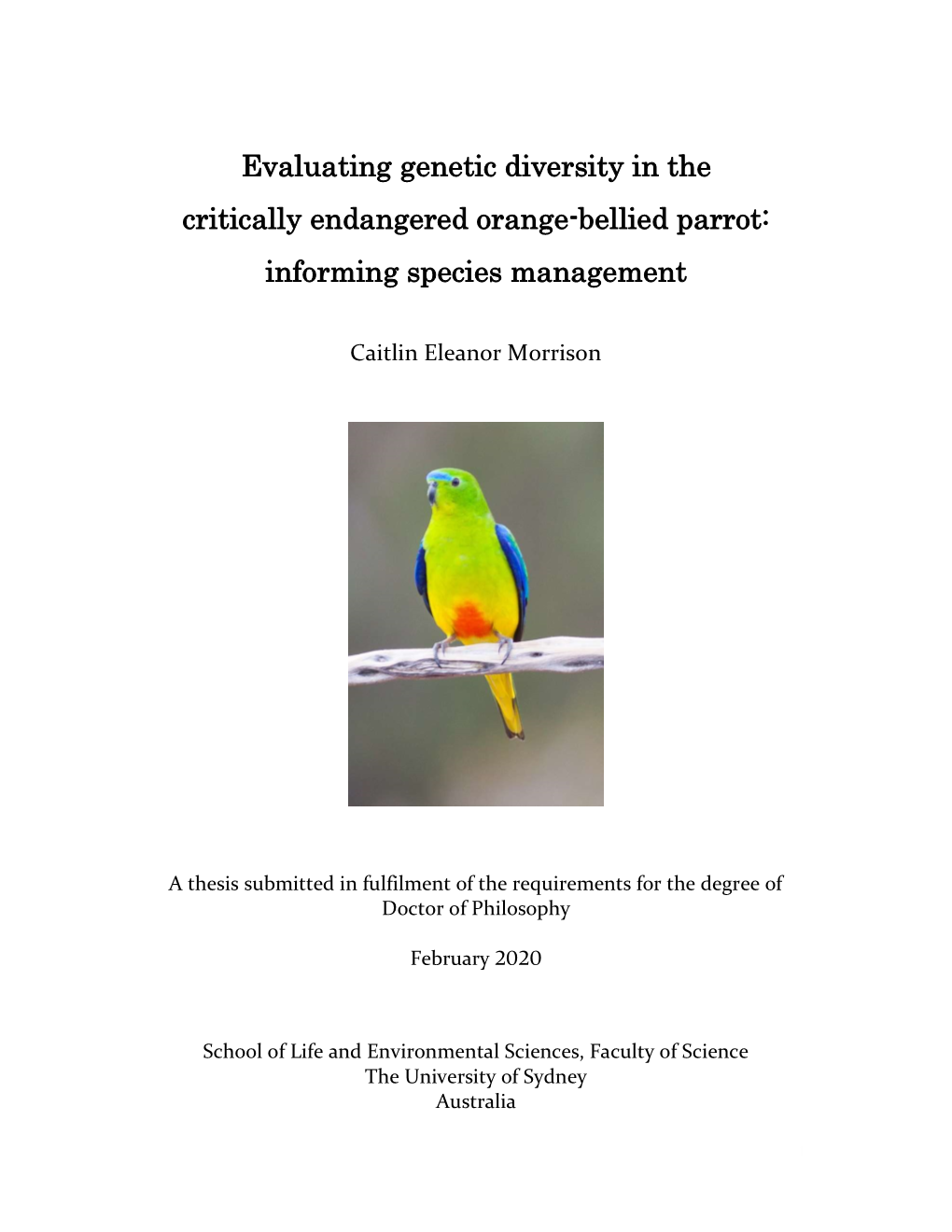
Load more
Recommended publications
-
Reproductive Success and Demography of the Orange-Bellied Parrot Neophema Chrysogaster
Reproductive success and demography of the Orange‐bellied Parrot Neophema chrysogaster Mark Christopher Holdsworth Submitted in fulfilment of the requirements for the Degree of Master of Science University of Tasmania, November 2006 Statement of originality This thesis does not contain any material accepted for a degree or diploma by this University or any other institution. To the best of my knowledge, no other personʹs published or otherwise written work has been used in this thesis without appropriate acknowledgment in the text. Mark Christopher Holdsworth Statement of authority of access This thesis may be reproduced, archived, and communicated in any material form in whole or in part by the University of Tasmania or its agents, and may be made available for loan and copying in accordance with the Copyright Act 1968. Mark Christopher Holdsworth i Orange‐bellied Parrots at Melaleuca (original watercolour by Mel Hills) ii Abstract The Orange‐bellied Parrot is one of only two obligate migratory parrots in the world. The species is listed nationally as endangered and has been the subject of intensive study and conservation activities over the past 25 years. Reproductive and demographic data collected over this period from the wild population form the basis of this thesis. Remote breeding sites in southwestern Tasmania at Melaleuca and Birchs Inlet were used to study this species in the wild. Through deployment of up to 52 artificial nest boxes and observations of natural nests at Melaleuca it was possible to collect information on a range of reproductive success parameters over a long period, including 12 consecutive breeding seasons. -
CITES Hooded Parrot Review
Original language: English AC28 Doc. 20.3.5 CONVENTION ON INTERNATIONAL TRADE IN ENDANGERED SPECIES OF WILD FAUNA AND FLORA ___________________ Twenty-eighth meeting of the Animals Committee Tel Aviv (Israel), 30 August-3 September 2015 Interpretation and implementation of the Convention Species trade and conservation Periodic review of species included in Appendices I and II [Resolution Conf 14.8 (Rev CoP16)] PERIODIC REVIEW OF PSEPHOTUS DISSIMILIS 1. This document has been submitted by Australia.* 2. After the 25th meeting of the Animals Committee (Geneva, July 2011) and in response to Notification to the Parties No. 2011/038, Australia committed to the evaluation of Psephotus dissimilis as part of the Periodic review of the species included in the CITES Appendices. 3. This taxon is endemic to Australia. 4. Following our review of the status of this species, Australia recommends to maintain Psephotus dissimilis on CITES Appendix I, in accordance with provisions of Resolution Conf. 9.24 (Rev CoP 16) to allow for further review. * The geographical designations employed in this document do not imply the expression of any opinion whatsoever on the part of the CITES Secretariat (or the United Nations Environment Programme) concerning the legal status of any country, territory, or area, or concerning the delimitation of its frontiers or boundaries. The responsibility for the contents of the document rests exclusively with its author. AC28 Doc. 20.3.5 – p. 1 AC28 Doc. 20.3.5 Annex CONVENTION ON INTERNATIONAL TRADE IN ENDANGERED SPECIES OF WILD FAUNA AND FLORA ____________________ DRAFT PROPOSAL TO AMEND THE APPENDICES (in accordance with Annex 4 to Resolution Conf. -
TAG Operational Structure
PARROT TAXON ADVISORY GROUP (TAG) Regional Collection Plan 5th Edition 2020-2025 Sustainability of Parrot Populations in AZA Facilities ...................................................................... 1 Mission/Objectives/Strategies......................................................................................................... 2 TAG Operational Structure .............................................................................................................. 3 Steering Committee .................................................................................................................... 3 TAG Advisors ............................................................................................................................... 4 SSP Coordinators ......................................................................................................................... 5 Hot Topics: TAG Recommendations ................................................................................................ 8 Parrots as Ambassador Animals .................................................................................................. 9 Interactive Aviaries Housing Psittaciformes .............................................................................. 10 Private Aviculture ...................................................................................................................... 13 Communication ........................................................................................................................ -
Parrots in the Wild
Magazine of the World Parrot Trust May 2002 No.51 PsittaScene PsittaSceneParrots in the Wild Kakapo chicks in the nest (Strigops habroptilus) Photo by DON MERTON The most productive season since Kakapo have been intensively managed, 26 chicks had hatched by April. The female called Flossie Members’had two. Seen here are twoExpedition! young she hatched in February 1998. Our report on page 16 describes how she feeds her chicks 900 rimu fruits at each feed - at least four times every night! Supporting parrot conservation in the wild and promoting parrot welfare in captivity. Printed by Brewers of Helston Ltd. Tel: 01326 558000. ‘psittacine’ (pronounced ‘sit a sin’) meaning ‘belonging or allied to the parrots’ or ‘parrot-like’ 0 PsittaPsitta African Grey Parrot SceneScene Trade in Cameroon Lobeke National Park Editor By ANASTASIA NGENYI, Volunteer Biologist, Rosemary Low, WWF Jengi SE Forest Project, BP 6776, Yaounde, Cameroon Glanmor House, Hayle, Cornwall, The forest region of Lobeke in the Southeast corner of Cameroon has TR27 4HB, UK been the focus of attention over the past decade at national and international level, owing to its rich natural resource. Its outstanding conservation importance is due to its abundance of Anastasia Ngenyi. fauna and the rich variety of commercial tree species. Natural CONTENTS resources in the area face numerous threats due to the increased demand in resource exploitation by African Grey Parrot Trade ..................2-3 the local communities and commercial pressure owing to logging and poaching for the bush meat trade. Palm Sunday Success ............................4 The area harbours an unusually high density of could generate enormous revenue that most likely Conservation Beyond the Cage ..............5 forest mammals' particularly so-called "charismatic would surpass present income from illegal trade in Palm Cockatoo Conservation ..............6-7 megafauna" such as elephants, gorillas and parrots. -
Habitat Types
Habitat Types The following section features ten predominant habitat types on the West Coast of the Eyre Peninsula, South Australia. It provides a description of each habitat type and the native plant and fauna species that commonly occur there. The fauna species lists in this section are not limited to the species included in this publication and include other coastal fauna species. Fauna species included in this publication are printed in bold. Information is also provided on specific threats and reference sites for each habitat type. The habitat types presented are generally either characteristic of high-energy exposed coastline or low-energy sheltered coastline. Open sandy beaches, non-vegetated dunefields, coastal cliffs and cliff tops are all typically found along high energy, exposed coastline, while mangroves, sand flats and saltmarsh/samphire are characteristic of low energy, sheltered coastline. Habitat Types Coastal Dune Shrublands NATURAL DISTRIBUTION shrublands of larger vegetation occur on more stable dunes and Found throughout the coastal environment, from low beachfront cliff-top dunes with deep stable sand. Most large dune shrublands locations to elevated clifftops, wherever sand can accumulate. will be composed of a mosaic of transitional vegetation patches ranging from bare sand to dense shrub cover. DESCRIPTION This habitat type is associated with sandy coastal dunes occurring The understory generally consists of moderate to high diversity of along exposed and sometimes more sheltered coastline. Dunes are low shrubs, sedges and groundcovers. Understory diversity is often created by the deposition of dry sand particles from the beach by driven by the position and aspect of the dune slope. -
Australia: from the Wet Tropics to the Outback Custom Tour Trip Report
AUSTRALIA: FROM THE WET TROPICS TO THE OUTBACK CUSTOM TOUR TRIP REPORT 4 – 20 OCTOBER 2018 By Andy Walker We enjoyed excellent views of Little Kingfisher during the tour. www.birdingecotours.com [email protected] 2 | TRIP REPORT Australia: From the Wet Tropics to the Outback, October 2018 Overview This 17-day customized Australia group tour commenced in Cairns, Queensland, on the 4th of October 2018 and concluded in Melbourne, Victoria, on the 20th of October 2018. The tour included a circuit around the Atherton Tablelands and surroundings from Cairns, a boat trip along the Daintree River, and a boat trip to the Great Barrier Reef (with snorkeling), a visit to the world- famous O’Reilly’s Rainforest Retreat in southern Queensland after a short flight to Brisbane, and rounded off with a circuit from Melbourne around the southern state of Victoria (and a brief but rewarding venture into southern New South Wales). The tour connected with many exciting birds and yielded a long list of eastern Australian birding specialties. Highlights of our time in Far North Queensland on the Cairns circuit included Southern Cassowary (a close male with chick in perfect light), hundreds of Magpie Geese, Raja Shelduck with young, Green and Cotton Pygmy Geese, Australian Brushturkey, Orange-footed Scrubfowl, Brown Quail, Squatter Pigeon, Wompoo, Superb, and perfect prolonged dawn- light views of stunning Rose-crowned Fruit Doves, displaying Australian Bustard, two nesting Papuan Frogmouths, White-browed Crake, Bush and Beach Stone-curlews (the latter -
Birdquest Australia (Western and Christmas
Chestnut-backed Button-quail in the north was a bonus, showing brilliantly for a long time – unheard of for this family (Andy Jensen) WESTERN AUSTRALIA 5/10 – 27 SEPTEMBER 2017 LEADER: ANDY JENSEN ASSISTANT: STUART PICKERING ! ! 1 BirdQuest Tour Report: Western Australia (including Christmas Island) 2017 www.birdquest-tours.com Western Shrike-tit was one of the many highlights in the southwest (Andy Jensen) Western Australia, if it were a country, would be the 10th largest in the world! The BirdQuest Western Australia (including Christmas Island) 2017 tour offered an unrivalled opportunity to cover a large portion of this area, as well as the offshore territory of Christmas Island (located closer to Indonesia than mainland Australia). Western Australia is a highly diverse region with a range of habitats. It has been shaped by the isolation caused by the surrounding deserts. This isolation has resulted in a richly diverse fauna, with a high degree of endemism. A must visit for any birder. This tour covered a wide range of the habitats Western Australia has to offer as is possible in three weeks, including the temperate Karri and Wandoo woodlands and mallee of the southwest, the coastal heathlands of the southcoast, dry scrub and extensive uncleared woodlands of the goldfields, coastal plains and mangroves around Broome, and the red-earth savannah habitats and tropical woodland of the Kimberley. The climate varied dramatically Conditions ranged from minus 1c in the Sterling Ranges where we were scraping ice off the windscreen, to nearly 40c in the Kimberley, where it was dust needing to be removed from the windscreen! We were fortunate with the weather – aside from a few minutes of drizzle as we staked out one of the skulkers in the Sterling Ranges, it remained dry the whole time. -
New Caledonia, Fiji & Vanuatu
Field Guides Tour Report Part I: New Caledonia Sep 5, 2011 to Sep 15, 2011 Phil Gregory The revamped tour was a little later this year and it seemed to make some things a bit easier, note how well we did with the rare Crow Honeyeater, and Kagu was as ever a standout. One first-year bird was rewarded with a nice juicy scorpion that our guide found, and this really is a fabulous bird to see, another down on Harlan's famiy quest, too, as an added bonus to what is a quite unique bird. Cloven-feathered Dove was also truly memorable, and watching one give that strange, constipated hooting call was fantastic and this really is one of the world's best pigeons. Air Calin did their best to make life hard with a somewhat late flight to Lifou, and I have to say the contrast with the Aussie pilots in Vanuatu was remarkable -- these French guys must still be learning as they landed the ATR 42's so hard and had to brake so fiercely! Still, it all worked out and the day trip for the Ouvea Parakeet worked nicely, whilst the 2 endemic white-eyes on Lifou were got really early for once. Nice food, an interesting Kanak culture, with a trip to the amazing Renzo Piano-designed Tjibaou Cultural Center also feasible this The fantastic Kagu, star of the tour! (Photo by guide Phil year, and a relaxed pace make this a fun birding tour with some Gregory) terrific endemic birds as a bonus. My thanks to Karen at the Field Guides office for hard work on the complex logistics for this South Pacific tour, to the very helpful Armstrong at Arc en Ciel, Jean-Marc at Riviere Bleue, and to Harlan and Bart for helping me with my bags when I had a back problem. -
Elegant Parrot
Elegant Parrot FAMILY: Psittacidae GENUS: Neophema SPECIES: elegans OTHER NAMES: Elegant Grass-parakeet, Grass-parakeet. Description: Small parrot displaying little sexual dimorphism. Male's crown, nape and uppers golden- olive. Blue band on forehead (extending beyond the eye) with pale blue line above it. Yellow between eye and beak. Wings olive with dark blue on margin. Turquoise shoulder patch (narrow). Flight feathers deep blue, central tail feathers dull blue, outers blue edged with yellow. Underparts including underside of tail yellow. Adult females resemble males but is duller in colour on upper parts and lacks golden tinge. Flight feathers brown. Immatures are duller versions of the adults and lack the band on forehead. Young cocks are usually more yellow on underparts than hens, and young hens usually have a pale stripe under the wings which disappears after the first moult. The Elegant Parrot is most often encountered in flocks of 20-100 or more, except in the breeding season when tend to be found either in pairs or small parties. It, like other Neophemas, is quiet, unobtrusive and forages almost entirely on the ground. Its flight is high, swift and direct. Partly nomadic, the Elegant Parrot may be encountered in the company of the Blue-winged Parrot. Length: 225mm. Subspecies: None. Distribution: From south-western NSW to the Mt Lofty Ranges and Kangaroo Island in SA and in WA from Esperance to Perth. Habitat: Heathland and open country, open woodland, cropland and semiarid scrub. Diet: Seeds of grasses and herbacious plants. Breeding: August-January. The usual nesting site is a small tree cavity near the ground. -
Aesthetics, Economics and Conservation of the Endangered Orange-Bellied Parrot
Agenda, Volume 7, Number 2, 2000, pages 153-166 Aesthetics, Economics and Conservation of the Endangered Orange-Bellied Parrot Harry Clarke r T l h e Orange-bellied Parrot (N. chrysogaster), or OBP, is an exceedingly rare Australian parrot and, indeed, one of the world’s rarest birds. The genus X Neophema to which it belongs comprise small, graceful grass parrots in the family Psittacidae that include both parrots and lorikeets. They are ground dwelling and southern-based Australian parrots. Apart from the OBP, members include the Turquoise Parrot (N. pulchella), the Scarlet-chested Parrot (N. splendida), the Blue-winged Parrot (N. chrysostoma), the Elegant Parrot (N. elegans) and the Rock Parrot (N. petrophila). Members of this genus are described by Trounson (1996:54) as ‘among the most beautiful of birds’. All are endemic to Australia and none, except the Blue-winged Parrot, are common with the OBP being by far the most rare. Bourke’s Parrot (N. bourkii) formerly classified with this genus, is now placed in a different genus (Christidis and Boles, 1994). The OBP breeds in summer on the south-west coast of Tasmania in hollow bearing eucalypts which grow adjacent to the buttongrass plains where it feeds. On completion of its breeding season, the population migrates north across Bass Strait via King Island during March-April. Most OBPs then reside in the south east region of Australia between Gippsland, eastern Victoria and The Coorong in south-eastern South Australia. Particular concentrations of OBPs are found in the saltmarshes of Port Phillip Bay (Forshaw (1989:284) claims up to 70 per cent live in this habitat) where they feed on salt-resistant plants restricted to this habitat (Loyn et al, 1986). -
Native Animal Species List
Native animal species list Native animals in South Australia are categorised into one of four groups: • Unprotected • Exempt • Basic • Specialist. To find out the category your animal is in, please check the list below. However, Specialist animals are not listed. There are thousands of them, so we don’t carry a list. A Specialist animal is simply any native animal not listed in this document. Mammals Common name Zoological name Species code Category Dunnart Fat-tailed dunnart Sminthopsis crassicaudata A01072 Basic Dingo Wild dog Canis familiaris Not applicable Unprotected Gliders Squirrel glider Petaurus norfolcensis E04226 Basic Sugar glider Petaurus breviceps E01138 Basic Possum Common brushtail possum Trichosurus vulpecula K01113 Basic Potoroo and bettongs Brush-tailed bettong (Woylie) Bettongia penicillata ogilbyi M21002 Basic Long-nosed potoroo Potorous tridactylus Z01175 Basic Rufous bettong Aepyprymnus rufescens W01187 Basic Rodents Mitchell's hopping-mouse Notomys mitchellii Y01480 Basic Plains mouse (Rat) Pseudomys australis S01469 Basic Spinifex hopping-mouse Notomys alexis K01481 Exempt Wallabies Parma wallaby Macropus parma K01245 Basic Red-necked pademelon Thylogale thetis Y01236 Basic Red-necked wallaby Macropus rufogriseus K01261 Basic Swamp wallaby Wallabia bicolor E01242 Basic Tammar wallaby Macropus eugenii eugenii C05889 Basic Tasmanian pademelon Thylogale billardierii G01235 Basic 1 Amphibians Common name Zoological name Species code Category Southern bell frog Litoria raniformis G03207 Basic Smooth frog Geocrinia laevis -
Of Parrots 3 Other Major Groups of Parrots 16
ONE What are the Parrots and Where Did They Come From? The Evolutionary History of the Parrots CONTENTS The Marvelous Diversity of Parrots 3 Other Major Groups of Parrots 16 Reconstructing Evolutionary History 5 Box 1. Ancient DNA Reveals the Evolutionary Relationships of the Fossils, Bones, and Genes 5 Carolina Parakeet 19 The Evolution of Parrots 8 How and When the Parrots Diversified 25 Parrots’ Ancestors and Closest Some Parrot Enigmas 29 Relatives 8 What Is a Budgerigar? 29 The Most Primitive Parrot 13 How Have Different Body Shapes Evolved in The Most Basal Clade of Parrots 15 the Parrots? 32 THE MARVELOUS DIVERSITY OF PARROTS The parrots are one of the most marvelously diverse groups of birds in the world. They daz- zle the beholder with every color in the rainbow (figure 3). They range in size from tiny pygmy parrots weighing just over 10 grams to giant macaws weighing over a kilogram. They consume a wide variety of foods, including fruit, seeds, nectar, insects, and in a few cases, flesh. They produce large repertoires of sounds, ranging from grating squawks to cheery whistles to, more rarely, long melodious songs. They inhabit a broad array of habitats, from lowland tropical rainforest to high-altitude tundra to desert scrubland to urban jungle. They range over every continent but Antarctica, and inhabit some of the most far-flung islands on the planet. They include some of the most endangered species on Earth and some of the most rapidly expanding and aggressive invaders of human-altered landscapes. Increasingly, research into the lives of wild parrots is revealing that they exhibit a corresponding variety of mating systems, communication signals, social organizations, mental capacities, and life spans.