CD93 Promotes Integrin-Β1 Activation and Fibronectin Fibrillogenesis During Tumor Angiogenesis
Total Page:16
File Type:pdf, Size:1020Kb
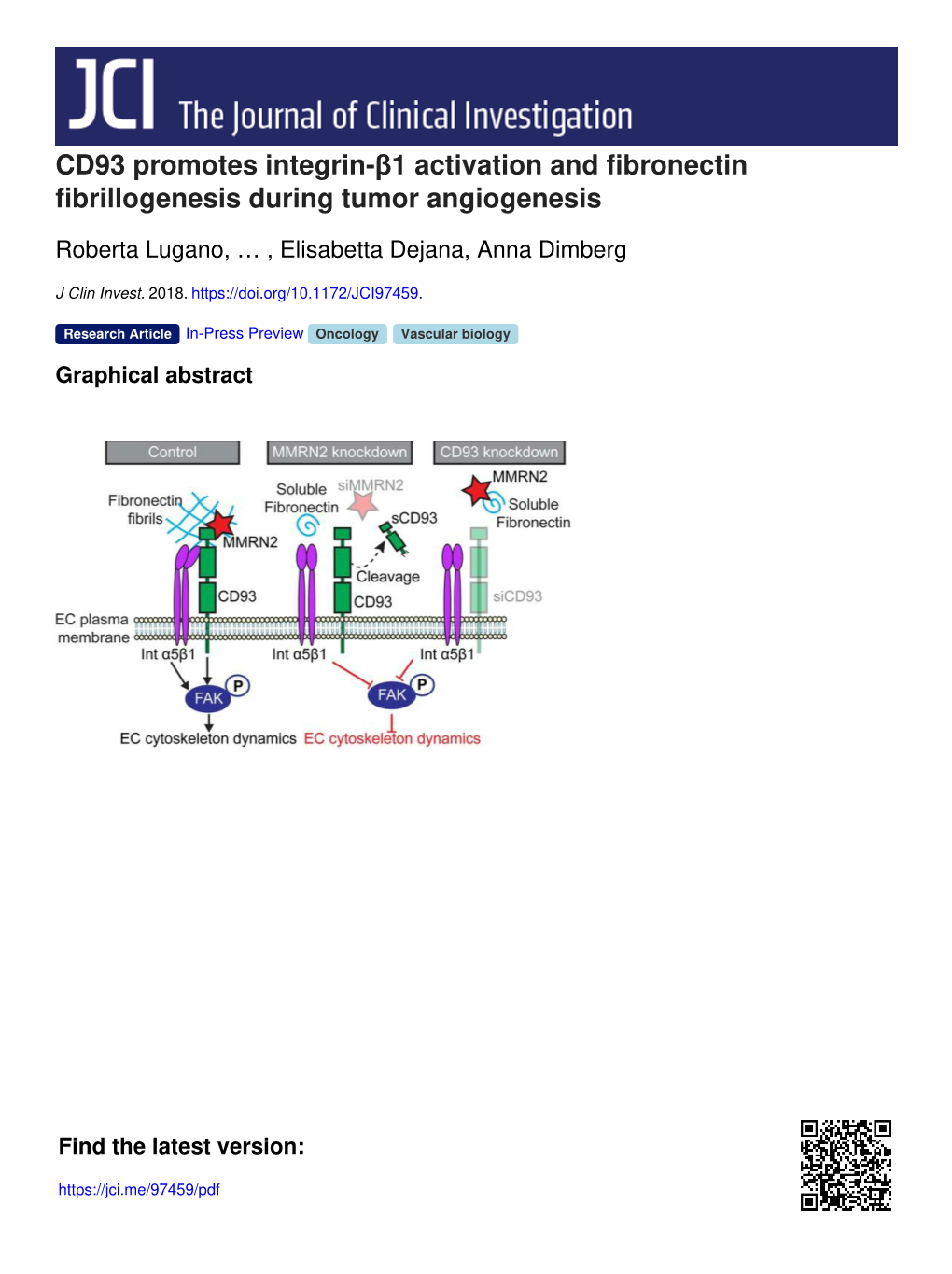
Load more
Recommended publications
-
Human and Mouse CD Marker Handbook Human and Mouse CD Marker Key Markers - Human Key Markers - Mouse
Welcome to More Choice CD Marker Handbook For more information, please visit: Human bdbiosciences.com/eu/go/humancdmarkers Mouse bdbiosciences.com/eu/go/mousecdmarkers Human and Mouse CD Marker Handbook Human and Mouse CD Marker Key Markers - Human Key Markers - Mouse CD3 CD3 CD (cluster of differentiation) molecules are cell surface markers T Cell CD4 CD4 useful for the identification and characterization of leukocytes. The CD CD8 CD8 nomenclature was developed and is maintained through the HLDA (Human Leukocyte Differentiation Antigens) workshop started in 1982. CD45R/B220 CD19 CD19 The goal is to provide standardization of monoclonal antibodies to B Cell CD20 CD22 (B cell activation marker) human antigens across laboratories. To characterize or “workshop” the antibodies, multiple laboratories carry out blind analyses of antibodies. These results independently validate antibody specificity. CD11c CD11c Dendritic Cell CD123 CD123 While the CD nomenclature has been developed for use with human antigens, it is applied to corresponding mouse antigens as well as antigens from other species. However, the mouse and other species NK Cell CD56 CD335 (NKp46) antibodies are not tested by HLDA. Human CD markers were reviewed by the HLDA. New CD markers Stem Cell/ CD34 CD34 were established at the HLDA9 meeting held in Barcelona in 2010. For Precursor hematopoetic stem cell only hematopoetic stem cell only additional information and CD markers please visit www.hcdm.org. Macrophage/ CD14 CD11b/ Mac-1 Monocyte CD33 Ly-71 (F4/80) CD66b Granulocyte CD66b Gr-1/Ly6G Ly6C CD41 CD41 CD61 (Integrin b3) CD61 Platelet CD9 CD62 CD62P (activated platelets) CD235a CD235a Erythrocyte Ter-119 CD146 MECA-32 CD106 CD146 Endothelial Cell CD31 CD62E (activated endothelial cells) Epithelial Cell CD236 CD326 (EPCAM1) For Research Use Only. -
NKG2D Promotes B1a Cell Development and Protection
NKG2D Promotes B1a Cell Development and Protection against Bacterial Infection Maja Lenartic, Vedrana Jelencic, Biljana Zafirova, Mateja Ozanic, Valentina Marecic, Slaven Jurkovic, Veronika Sexl, This information is current as Marina Santic, Felix M. Wensveen and Bojan Polic of September 25, 2021. J Immunol 2017; 198:1531-1542; Prepublished online 13 January 2017; doi: 10.4049/jimmunol.1600461 http://www.jimmunol.org/content/198/4/1531 Downloaded from Supplementary http://www.jimmunol.org/content/suppl/2017/01/12/jimmunol.160046 Material 1.DCSupplemental http://www.jimmunol.org/ References This article cites 45 articles, 18 of which you can access for free at: http://www.jimmunol.org/content/198/4/1531.full#ref-list-1 Why The JI? Submit online. • Rapid Reviews! 30 days* from submission to initial decision by guest on September 25, 2021 • No Triage! Every submission reviewed by practicing scientists • Fast Publication! 4 weeks from acceptance to publication *average Subscription Information about subscribing to The Journal of Immunology is online at: http://jimmunol.org/subscription Permissions Submit copyright permission requests at: http://www.aai.org/About/Publications/JI/copyright.html Email Alerts Receive free email-alerts when new articles cite this article. Sign up at: http://jimmunol.org/alerts The Journal of Immunology is published twice each month by The American Association of Immunologists, Inc., 1451 Rockville Pike, Suite 650, Rockville, MD 20852 Copyright © 2017 by The American Association of Immunologists, Inc. All rights reserved. Print ISSN: 0022-1767 Online ISSN: 1550-6606. The Journal of Immunology NKG2D Promotes B1a Cell Development and Protection against Bacterial Infection Maja Lenartic´,* Vedrana Jelencic´,* Biljana Zafirova,*,† Mateja Ozanic,‡ Valentina Marecic´,‡ Slaven Jurkovic´,x Veronika Sexl,{ Marina Santic ´,‡ Felix M. -
The Chondroitin Sulfate Moiety Mediates Thrombomodulin-Enhanced Adhesion and Migration of Vascular Smooth Muscle Cells
Pai et al. Journal of Biomedical Science (2018)25:14 https://doi.org/10.1186/s12929-018-0415-7 RESEARCH Open Access The chondroitin sulfate moiety mediates thrombomodulin-enhanced adhesion and migration of vascular smooth muscle cells Vincent Chunpeng Pai1†, I-Chung Lo2†, Yan wun Huang1, I-Ching Tsai1, Hui-Pin Cheng1, Guey-Yueh Shi2,3, Hua-Lin Wu2,3 and Meei Jyh Jiang1,2* Abstract Background: Thrombomodulin (TM), a transmembrane glycoprotein highly expressed in endothelial cells (ECs), is a potent anticoagulant maintaining circulation homeostasis. Under inflammatory states, TM expression is drastically reduced in ECs while vascular smooth muscle cells (VSMCs) show a robust expression of TM. The functional role of TM in VSMCs remains elusive. Methods: We examined the role of TM in VSMCs activities in human aortic VSMCs stimulated with platelet-derived growth factor-BB (PDGF-BB). Using rat embryonic aorta-derived A7r5 VSMCs which do not express TM, the role of the chondroitin sulfate (CS) moiety of TM in VSMCs was delineated with cells expressing wild-type TM and the CS-devoid TM mutant. Results: Expression of TM enhanced cell migration and adhesion/spreading onto type I collagen, but had no effect on cell proliferation. Knocking down TM with short hairpin RNA reduced PDGF-stimulated adhesion and migration of human aortic VSMCs. In A7r5 cells, TM-mediated cell adhesion was eradicated by pretreatment with chondroitinase ABC which degrades CS moiety. Furthermore, the TM mutant (TMS490, 492A)devoidofCS moiety failed to increase cell adhesion, spreading or migration. Wild-type TM, but not TMS490, 492A,increased focal adhesion kinase (FAK) activation during cell adhesion, and TM-enhanced cell migration was abolished by a function-blocking anti-integrin β1 antibody. -
CD93 and Dystroglycan Cooperation in Human Endothelial Cell Adhesion and Migration
www.impactjournals.com/oncotarget/ Oncotarget, Vol. 7, No. 9 CD93 and dystroglycan cooperation in human endothelial cell adhesion and migration Federico Galvagni1,*, Federica Nardi1,*, Marco Maida1, Giulia Bernardini1, Silvia Vannuccini2, Felice Petraglia2, Annalisa Santucci1, Maurizio Orlandini1 1 Department of Biotechnology, Chemistry and Pharmacy, University of Siena, 2-53100 Siena, Italy 2 Department of Molecular and Developmental Medicine, Obstetrics and Gynecology, University of Siena, 53100 Siena, Italy *These authors contributed equally to this work Correspondence to: Maurizio Orlandini, e-mail: [email protected] Keywords: angiogenesis, signal transduction, C1qRp, Src, Cbl Received: June 29, 2015 Accepted: January 22, 2016 Published: February 02, 2016 ABSTRACT CD93 is a transmembrane glycoprotein predominantly expressed in endothelial cells. Although CD93 displays proangiogenic activity, its molecular function in angiogenesis still needs to be clarified. To get molecular insight into the biological role of CD93 in the endothelium, we performed proteomic analyses to examine changes in the protein profile of endothelial cells after CD93 silencing. Among differentially expressed proteins, we identified dystroglycan, a laminin-binding protein involved in angiogenesis, whose expression is increased in vascular endothelial cells within malignant tumors. Using immunofluorescence, FRET, and proximity ligation analyses, we observed a close interaction between CD93 and β-dystroglycan. Moreover, silencing experiments showed that CD93 and dystroglycan promoted endothelial cell migration and organization into capillary-like structures. CD93 proved to be phosphorylated on tyrosine 628 and 644 following cell adhesion on laminin through dystroglycan. This phosphorylation was shown to be necessary for a proper endothelial migratory phenotype. Moreover, we showed that during cell spreading phosphorylated CD93 recruited the signaling protein Cbl, which in turn was phosphorylated on tyrosine 774. -
Global H3k4me3 Genome Mapping Reveals Alterations of Innate Immunity Signaling and Overexpression of JMJD3 in Human Myelodysplastic Syndrome CD34 Þ Cells
Leukemia (2013) 27, 2177–2186 & 2013 Macmillan Publishers Limited All rights reserved 0887-6924/13 www.nature.com/leu ORIGINAL ARTICLE Global H3K4me3 genome mapping reveals alterations of innate immunity signaling and overexpression of JMJD3 in human myelodysplastic syndrome CD34 þ cells YWei1, R Chen2, S Dimicoli1, C Bueso-Ramos3, D Neuberg4, S Pierce1, H Wang2, H Yang1, Y Jia1, H Zheng1, Z Fang1, M Nguyen3, I Ganan-Gomez1,5, B Ebert6, R Levine7, H Kantarjian1 and G Garcia-Manero1 The molecular bases of myelodysplastic syndromes (MDS) are not fully understood. Trimethylated histone 3 lysine 4 (H3K4me3) is present in promoters of actively transcribed genes and has been shown to be involved in hematopoietic differentiation. We performed a genome-wide H3K4me3 CHIP-Seq (chromatin immunoprecipitation coupled with whole genome sequencing) analysis of primary MDS bone marrow (BM) CD34 þ cells. This resulted in the identification of 36 genes marked by distinct higher levels of promoter H3K4me3 in MDS. A majority of these genes are involved in nuclear factor (NF)-kB activation and innate immunity signaling. We then analyzed expression of histone demethylases and observed significant overexpression of the JmjC-domain histone demethylase JMJD3 (KDM6b) in MDS CD34 þ cells. Furthermore, we demonstrate that JMJD3 has a positive effect on transcription of multiple CHIP-Seq identified genes involved in NF-kB activation. Inhibition of JMJD3 using shRNA in primary BM MDS CD34 þ cells resulted in an increased number of erythroid colonies in samples isolated from patients with lower-risk MDS. Taken together, these data indicate the deregulation of H3K4me3 and associated abnormal activation of innate immunity signals have a role in the pathogenesis of MDS and that targeting these signals may have potential therapeutic value in MDS. -
Capacity of Human Dendritic Cells Uptake Receptor Expression And
The Novel Cyclophilin-Binding Drug Sanglifehrin A Specifically Affects Antigen Uptake Receptor Expression and Endocytic Capacity of Human Dendritic Cells This information is current as of September 25, 2021. Andrea M. Woltman, Nicole Schlagwein, Sandra W. van der Kooij and Cees van Kooten J Immunol 2004; 172:6482-6489; ; doi: 10.4049/jimmunol.172.10.6482 http://www.jimmunol.org/content/172/10/6482 Downloaded from References This article cites 44 articles, 20 of which you can access for free at: http://www.jimmunol.org/content/172/10/6482.full#ref-list-1 http://www.jimmunol.org/ Why The JI? Submit online. • Rapid Reviews! 30 days* from submission to initial decision • No Triage! Every submission reviewed by practicing scientists • Fast Publication! 4 weeks from acceptance to publication by guest on September 25, 2021 *average Subscription Information about subscribing to The Journal of Immunology is online at: http://jimmunol.org/subscription Permissions Submit copyright permission requests at: http://www.aai.org/About/Publications/JI/copyright.html Email Alerts Receive free email-alerts when new articles cite this article. Sign up at: http://jimmunol.org/alerts The Journal of Immunology is published twice each month by The American Association of Immunologists, Inc., 1451 Rockville Pike, Suite 650, Rockville, MD 20852 Copyright © 2004 by The American Association of Immunologists All rights reserved. Print ISSN: 0022-1767 Online ISSN: 1550-6606. The Journal of Immunology The Novel Cyclophilin-Binding Drug Sanglifehrin A Specifically Affects Antigen Uptake Receptor Expression and Endocytic Capacity of Human Dendritic Cells1 Andrea M. Woltman,2 Nicole Schlagwein, Sandra W. van der Kooij, and Cees van Kooten Sanglifehrin A (SFA) is a recently developed immunosuppressant that belongs to the family of immunophilin-binding ligands. -
Endotoxin Enhances Tissue Factor and Suppresses Thrombomodulin Expression of Human Vascular Endothelium in Vitro Kevin L
Endotoxin Enhances Tissue Factor and Suppresses Thrombomodulin Expression of Human Vascular Endothelium In Vitro Kevin L. Moore,* Sharon P. Andreoli,* Naomi L. Esmon,1 Charles T. Esmon,l and Nils U. Bang1l Department ofMedicine, Section ofHematology/Oncology,* and Department ofPediatrics, Section ofNephrology,t Indiana University School ofMedicine, Indianapolis, Indiana 46223; Oklahoma Medical Research Foundation,§ Oklahoma City, Oklahoma 73104; and Lilly Laboratory for Clinical Research,1' Indianapolis, Indiana 46202 Abstract to support the assembly of clotting factor complexes on their surfaces (13, 14). Endotoxemia is frequently associated clinically with disseminated Under physiologic conditions the thromboresistant properties intravascular coagulation (DIC); however, the mechanism of en- ofthe endothelium are predominant. In some pathological states, dotoxin action in vivo is unclear. Modulation of tissue factor however, this may not be the case. Gram-negative sepsis is fre- (TF) and thrombomodulin (TM) expression on the endothelial quently associated with varying degrees of disseminated intra- surface may be relevant pathophysiologic mechanisms. Stimu- vascular coagulation (DIC),' which is thought to be triggered by lation of human umbilical vein endothelial cells with endotoxin endotoxemia. The pathophysiology of DIC in gram-negative (1 ,ug/ml) increased surface TF activity from 1.52±0.84 to sepsis is complex and the mechanism(s) by which endotoxemia 11.89±8.12 mU/ml-106 cells at 6 h (n = 11) which returned to promotes intravascular coagulation in vivo is unclear. Recently, baseline by 24 h. Repeated stimulation at 24 h resulted in renewed reports by several investigators provide evidence that the throm- TF expression. Endotoxin (1 ,tg/ml) also caused a decrease in boresistance of the endothelial cell is diminished after exposure TM expression to 55.0±6.4% of control levels at 24 h (n = 10) to endotoxin in the absence of other cell types. -
Path Ggf 5 2020.Pdf
Hemostasis Hemostasis and Thrombosis Normal hemostasis is a consequence of tightly regulated processes that maintain blood in a fluid state in normal vessels, yet also permit the rapid formation of a hemostatic clot at the site of a vascular injury. Thrombosis involves blood clot formation within intact vessels. Both hemostasis and thrombosis involve three components: the vascular wall, platelets and the coagulation cascade. Elements of the Hemostatic process • Endothelium • Anti-thrombosis • Pro-thrombosis • Platelets • Platelet-endothelial cell interaction • Coagulation cascade http://www.as.miami.edu/chemistry/2086/chapter_21/NEW-Chap21_class_part1_files/image002.jpg After initial injury there is a brief period of arteriolar vasoconstriction mediated by reflex neurogenic mechanisms and augmented by the local secretion of factors such as endothelin (a potent endothelium-derived vasoconstrictor) The effect is transient, however, and bleeding would resume if not for activation of the platelet and coagulation systems. Endothelial injury exposes highly thrombogenic subendothelial extracellular matrix (ECM), facilitating platelet adherence and activation. Activation of platelets results in a dramatic shape change (from small rounded discs to flat plates with markedly increased surface area), as well as the release of secretory granules. Within minutes the secreted products recruit additional platelets (aggregation) to form a hemostatic plug; this process is referred to as primary hemostasis. http://www.ouhsc.edu/platelets/Platelet%20Pic s/Platelets3.jpg http://medcell.med.yale.edu/histology/blood_bone_marr ow_lab/images/platelets_em.jpg Tissue factor is also exposed at the site of injury. Also known as factor III and thromboplastin, tissue factor is a membrane-bound procoagulant glycoprotein synthesized by endothelial cells. It acts in conjunction with factor VII (see below) as the major in vivo initiator of the coagulation cascade, eventually culminating in thrombin generation. -
Proteomic Analysis of Uterine Fluid During the Pre-Implantation Period of Pregnancy in Cattle
REPRODUCTIONRESEARCH Proteomic analysis of uterine fluid during the pre-implantation period of pregnancy in cattle Niamh Forde, Paul A McGettigan, Jai P Mehta, Lydia O’Hara, Solomon Mamo, Fuller W Bazer1, Thomas E Spencer2 and Pat Lonergan School of Agriculture and Food Science, University College Dublin, Belfield, Dublin 4, Ireland, 1Department of Animal Science, Texas A&M University, College Station, Texas 77843-2471, USA and 2Department of Animal Sciences, Washington State University, Pullman, Washington 99164-6353, USA Correspondence should be addressed to N Forde; Email: [email protected] Abstract The aims of this study were i) to characterize the global changes in the composition of the uterine luminal fluid (ULF) from pregnant heifers during pregnancy recognition (day 16) using nano-LC MS/MS; ii) to describe quantitative changes in selected proteins in the ULF from days 10, 13, 16 and 19 by Isobaric tags for Relative and Absolute Quantification (iTRAQ) analysis; and iii) to determine whether these proteins are of endometrial or conceptus origin, by examining the expression profiles of the associated transcripts by RNA sequencing. On day 16, 1652 peptides were identified in the ULF by nano-LC MS/MS. Of the most abundant proteins present, iTRAQ analysis revealed that RPB4, TIMP2 and GC had the same expression pattern as IFNT, while the abundance of IDH1, CST6 and GDI2 decreased on either day 16 or 19. ALDOA, CO3, GSN, HSP90A1, SERPINA31 and VCN proteins decreased on day 13 compared with day 10 but subsequently increased on day 16 (P!0.05). Purine nucleoside phosphorylase (PNP) and HSPA8 decreased on day 13, increased on day 16 and decreased and increased on day 19 (P!0.05). -
Endothelial Cells Transcription in Rhoa and Fos Stimulates C- but Not ICAM
Intercellular Adhesion Molecule (ICAM)-1, But Not ICAM-2, Activates RhoA and Stimulates c-fos and rhoA Transcription in Endothelial Cells This information is current as of September 25, 2021. Paul W. Thompson, Anna M. Randi and Anne J. Ridley J Immunol 2002; 169:1007-1013; ; doi: 10.4049/jimmunol.169.2.1007 http://www.jimmunol.org/content/169/2/1007 Downloaded from References This article cites 38 articles, 19 of which you can access for free at: http://www.jimmunol.org/content/169/2/1007.full#ref-list-1 http://www.jimmunol.org/ Why The JI? Submit online. • Rapid Reviews! 30 days* from submission to initial decision • No Triage! Every submission reviewed by practicing scientists • Fast Publication! 4 weeks from acceptance to publication by guest on September 25, 2021 *average Subscription Information about subscribing to The Journal of Immunology is online at: http://jimmunol.org/subscription Permissions Submit copyright permission requests at: http://www.aai.org/About/Publications/JI/copyright.html Email Alerts Receive free email-alerts when new articles cite this article. Sign up at: http://jimmunol.org/alerts The Journal of Immunology is published twice each month by The American Association of Immunologists, Inc., 1451 Rockville Pike, Suite 650, Rockville, MD 20852 Copyright © 2002 by The American Association of Immunologists All rights reserved. Print ISSN: 0022-1767 Online ISSN: 1550-6606. The Journal of Immunology Intercellular Adhesion Molecule (ICAM)-1, But Not ICAM-2, Activates RhoA and Stimulates c-fos and rhoA Transcription in Endothelial Cells1 Paul W. Thompson,*† Anna M. Randi,‡ and Anne J. Ridley2*† ICAM-1 and -2 are integrin-binding Ig superfamily adhesion molecules that are important for leukocyte transmigration across endothelial monolayers. -
And Heterotypic Interaction of Merlin and Ezrin
Journal of Cell Science 112, 895-904 (1999) 895 Printed in Great Britain © The Company of Biologists Limited 1999 JCS0140 Homotypic and heterotypic interaction of the neurofibromatosis 2 tumor suppressor protein merlin and the ERM protein ezrin Mikaela Grönholm1,*, Markku Sainio1, Fang Zhao1, Leena Heiska1, Antti Vaheri2 and Olli Carpén1 Departments of 1Pathology and 2Virology, University of Helsinki, Haartman Institute, PO Box 21 (Haartmaninkatu 3), FIN-00014 Helsinki *Author for correspondence (e-mail: mikaela.gronholm@helsinki.fi) Accepted 23 December 1998; published on WWW 25 February 1999 SUMMARY Ezrin, radixin and moesin (ERM) are homologous proteins, involves interaction between the amino- and carboxy- which are linkers between plasma membrane components termini. The amino-terminal association domain of merlin and the actin-containing cytoskeleton. The ERM protein involves residues 1-339 and has similar features with the family members associate with each other in a homotypic amino-terminal association domain of ezrin. The carboxy- and heterotypic manner. The neurofibromatosis 2 (NF2) terminal association domain cannot be mapped as precisely tumor suppressor protein merlin (schwannomin) is as in ezrin, but it requires residues 585-595 and a more structurally related to ERM members. Merlin is involved amino-terminal segment. Unlike ezrin, merlin does not in tumorigenesis of NF2-associated and sporadic require activation for self-association but native merlin schwannomas and meningiomas, but the tumor suppressor molecules can interact with each other. Heterodimerization mechanism is poorly understood. We have studied the between merlin and ezrin, however, occurs only following ability of merlin to self-associate and bind ezrin. Ezrin was conformational alterations in both proteins. -
ERM Protein Family
Cell Biology 2018; 6(2): 20-32 http://www.sciencepublishinggroup.com/j/cb doi: 10.11648/j.cb.20180602.11 ISSN: 2330-0175 (Print); ISSN: 2330-0183 (Online) Structure and Functions: ERM Protein Family Divine Mensah Sedzro 1, †, Sm Faysal Bellah 1, 2, †, *, Hameed Akbar 1, Sardar Mohammad Saker Billah 3 1Laboratory of Cellular Dynamics, School of Life Science, University of Science and Technology of China, Hefei, China 2Department of Pharmacy, Manarat International University, Dhaka, Bangladesh 3Department of Chemistry, Govt. M. M. University College, Jessore, Bangladesh Email address: *Corresponding author † These authors contributed equally to this work To cite this article: Divine Mensah Sedzro, Sm Faysal Bellah, Hameed Akbar, Sardar Mohammad Saker Billah. Structure and Functions: ERM Protein Family. Cell Biology . Vol. 6, No. 2, 2018, pp. 20-32. doi: 10.11648/j.cb.20180602.11 Received : September 15, 2018; Accepted : October 6, 2018; Published : October 29, 2018 Abstract: Preservation of the structural integrity of the cell depends on the plasma membrane in eukaryotic cells. Interaction between plasma membrane, cytoskeleton and proper anchorage influence regular cellular processes. The needed regulated connection between the membrane and the underlying actin cytoskeleton is therefore made available by the ERM (Ezrin, Radixin, and Moesin) family of proteins. ERM proteins also afford the required environment for the diffusion of signals in reactions to extracellular signals. Other studies have confirmed the importance of ERM proteins in different mode organisms and in cultured cells to emphasize the generation and maintenance of specific domains of the plasma membrane. An essential attribute of almost all cells are the specialized membrane domains.