Studying the Molecular Dynamics of Deuterium Molecules Trapped Inside a Simple Clathrate Hydrate Using High Resolution Raman Spectroscopy
Total Page:16
File Type:pdf, Size:1020Kb
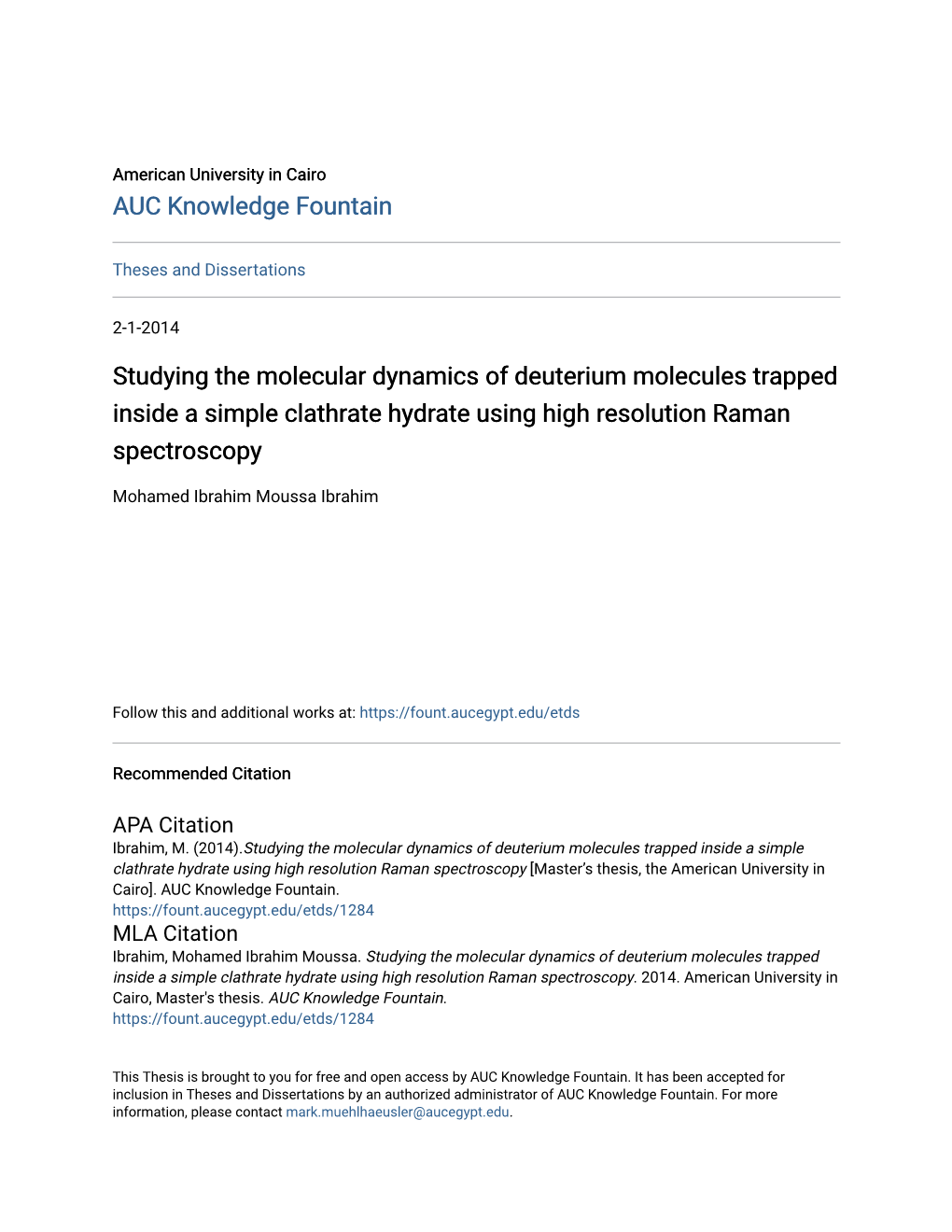
Load more
Recommended publications
-
Thermal Regeneration of Sulfuric Acid Hydrates After Irradiation ⇑ Mark J
Icarus 219 (2012) 561–566 Contents lists available at SciVerse ScienceDirect Icarus journal homepage: www.elsevier.com/locate/icarus Thermal regeneration of sulfuric acid hydrates after irradiation ⇑ Mark J. Loeffler , Reggie L. Hudson Astrochemistry Laboratory, NASA Goddard Space Flight Center, Mail Code 691, Greenbelt, MD 20771, United States article info abstract Article history: In an attempt to more completely understand the surface chemistry of the jovian icy satellites, we have Received 17 January 2012 investigated the effect of heating on two irradiated crystalline sulfuric acid hydrates, H2SO4Á4H2O and Revised 13 March 2012 H2SO4ÁH2O. At temperatures relevant to Europa and the warmer jovian satellites, post-irradiation heating Accepted 25 March 2012 recrystallized the amorphized samples and increased the intensities of the remaining hydrate’s infrared Available online 10 April 2012 absorptions. This thermal regeneration of the original hydrates was nearly 100% efficient, indicating that over geological times, thermally-induced phase transitions enhanced by temperature fluctuations will Keywords: reform a large fraction of crystalline hydrated sulfuric acid that is destroyed by radiation processing. Europa The work described is the first demonstration of the competition between radiation-induced amorphiza- Ices, IR spectroscopy Jupiter, Satellites tion and thermally-induced recrystallization in icy ionic solids relevant to the outer Solar System. Impact processes Published by Elsevier Inc. Cosmic rays 1. Introduction ic, salty, or acidic, could be transported to a surface by a variety of mechanisms (Kargel et al., 2000; Greenburg, 2010). Primordial sub- Remote sensing of Jupiter’s icy satellites has revealed that even surface SO2 (Noll et al., 1995) and CO2 (Moore et al., 1999) could though their surfaces are composed mostly of water ice (Kuiper, also be carried upward by geological processes. -
Toxicological Review of Chloral Hydrate (CAS No. 302-17-0) (PDF)
EPA/635/R-00/006 TOXICOLOGICAL REVIEW OF CHLORAL HYDRATE (CAS No. 302-17-0) In Support of Summary Information on the Integrated Risk Information System (IRIS) August 2000 U.S. Environmental Protection Agency Washington, DC DISCLAIMER This document has been reviewed in accordance with U.S. Environmental Protection Agency policy and approved for publication. Mention of trade names or commercial products does not constitute endorsement or recommendation for use. Note: This document may undergo revisions in the future. The most up-to-date version will be made available electronically via the IRIS Home Page at http://www.epa.gov/iris. ii CONTENTS—TOXICOLOGICAL REVIEW for CHLORAL HYDRATE (CAS No. 302-17-0) FOREWORD .................................................................v AUTHORS, CONTRIBUTORS, AND REVIEWERS ................................ vi 1. INTRODUCTION ..........................................................1 2. CHEMICAL AND PHYSICAL INFORMATION RELEVANT TO ASSESSMENTS ..... 2 3. TOXICOKINETICS RELEVANT TO ASSESSMENTS ............................3 4. HAZARD IDENTIFICATION ................................................6 4.1. STUDIES IN HUMANS - EPIDEMIOLOGY AND CASE REPORTS .................................................6 4.2. PRECHRONIC AND CHRONIC STUDIES AND CANCER BIOASSAYS IN ANIMALS ................................8 4.2.1. Oral ..........................................................8 4.2.2. Inhalation .....................................................12 4.3. REPRODUCTIVE/DEVELOPMENTAL STUDIES ..........................13 -
Hydrate Risk Assessment and Restart-Procedure Optimization Of
Hydrate Risk Assessment and Restart- Procedure Optimization of an Offshore Well Using a Transient Hydrate Prediction Model L.E. Zerpa, E.D. Sloan, C.A. Koh, and A.K. Sum, Colorado School of Mines Summary This paper focuses on the application of the gas-hydrate model A produced-hydrocarbon stream from a wellhead encounters for- to study the hydrate-plugging risk of an offshore well with a typ- mation of solid gas-hydrate deposits, which plug flowlines and ical geometry and fluid properties from the Caratinga field located which are one of the most challenging problems in deep subsea fa- in the Campos basin, Brazil. Three different periods of the well cilities. This paper describes a gas-hydrate model for oil-dominated life are considered: an early stage with low production gas/oil ratio systems, which can be used for the design and optimization of facil- (GOR) and low water cut, a middle stage with an increased GOR, ities focusing on the prevention, management, and remediation of and a late stage with higher GOR and higher water cut. The hy- hydrates in flowlines. Using a typical geometry and fluid properties drate-plugging risk is estimated from the calculation of three per- of an offshore well from the Caratinga field located in the Campos formance measures (pressure drop along flowline, hydrate volume basin in Brazil, the gas-hydrate model is applied to study the hy- fraction in pipe, and hydrate-slurry relative viscosity) in an attempt drate-plugging risk at three different periods of the well life. Addi- to quantify the plugging risk. -
Synthesis of Methane Hydrate from Ice Powder Accelerated by Doping
www.nature.com/scientificreports OPEN Synthesis of Methane Hydrate from Ice Powder Accelerated by Doping Ethanol into Methane Gas Received: 2 May 2019 Yen-An Chen1, Liang-Kai Chu1, Che-Kang Chu1, Ryo Ohmura2 & Li-Jen Chen 1,2 Accepted: 13 August 2019 Clathrate hydrate is considered to be a potential medium for gas storage and transportation. Slow Published: xx xx xxxx kinetics of hydrate formation is a hindrance to the commercialized process development of such applications. The kinetics of methane hydrate formation from the reaction of ice powder and methane gas doped with/without saturated ethanol vapor at constant pressure of 16.55 ± 0.20 MPa and constant temperature ranging from −15 to −1.0 °C were investigated. The methane hydrate formation can be dramatically accelerated by simply doping ethanol into methane gas with ultralow ethanol concentration (<94 ppm by mole fraction) in the gas phase. For ethanol-doped system 80.1% of ice powder were converted into methane hydrate after a reaction time of 4 h, while only 26.6% of ice powder was converted into methane hydrate after a reaction time of 24 h when pure methane gas was used. Furthermore, this trace amount of ethanol could also substantially suppress the self-preservation efect to enhance the dissociation rate of methane hydrate (operated at 1 atm and temperatures below the ice melting point). In other words, a trace amount of ethanol doped in methane gas can act as a kinetic promoter for both the methane hydrate formation and dissociation. Clathrate hydrates are ice-like nonstoichiometric crystalline compounds formed by guest molecules encapsulated in the hydrogen bonded water cages at elevated pressures and low temperatures1. -
Methanol—Inhibitor Or Promoter of the Formation of Gas Hydrates from Deuterated Ice?
BOBEV AND TAIT: METHANOL—INHIBITOR OR PROMOTER? 1209 American Mineralogist, Volume 89, pages 1208–1214, 2004 Methanol—inhibitor or promoter of the formation of gas hydrates from deuterated ice? SVILEN BOBEV* AND KIMBERLY T. TAIT Manuel Lujan Jr. Neutron Scattering Center, LANSCE 12 MS H805, Los Alamos National Laboratory, Los Alamos, New Mexico 87545, U.S.A. ABSTRACT Kinetic studies are reported of the effect of methanol on the rate of formation of CO2- and CH4-hy- drates by means of in situ time-of-flight neutron powder diffraction. The experiments were carried out at temperatures ranging from 200 to 250 K and pressures up to 7 MPa. The samples were prepared from mixtures of ground, deuterated ice and deuterated methanol (up to 20 vol%), which were transformed in situ into CO2- or CH4-hydrates by pressurizing the systems with the corresponding gas. The observed rates of formation of hydrates are orders of magnitude higher than the rate of formation from pure deuterated ice under the same pressure and temperature conditions. Glycols and alcohols, methanol in particular, are long known as thermodynamic inhibitors of hydrate formation. Our study indicates that methanol can also act as a kinetic promoter for the formation of gas hydrates. Preliminary data suggest that the kinetics also depend strongly on concentration and the isotopic composition. INTRODUCTION particular, time-dependent neutron diffraction at a variety of tem- The crystal structures, thermodynamic models, and engineer- peratures and pressures has been effectively used to research the ing applications of CO2- and CH4-hydrates have been extensively kinetics of gas hydrate formation and dissociation (Henning et al. -
Arxiv:1803.01452V1 [Astro-Ph.EP] 5 Mar 2018
The when and where of water in the history of the universe Karla de Souza Torres1, and Othon Cabo Winter2 1CEFET-MG, Curvelo, Brazil; E-mail: [email protected] 2UNESP, Grupo de Din^amica Orbital & Planetologia, Guaratinguet´a,Brazil E-mail: [email protected] Abstract It is undeniable that life as we know it depends on liquid water. It is difficult to imagine any biochemical machinery that does not require water. On Earth, life adapts to the most diverse environments and, once established, it is very resilient. Considering that water is a common compound in the Universe, it seems possible (maybe even likely) that one day we will find life elsewhere in the universe. In this study, we review the main aspects of water as an essential compound for life: when it appeared since the Big Bang, and where it spread throughout the diverse cosmic sites. Then, we describe the strong relation between water and life, as we know it. Keywords water; life; universe; H2O; astrobiology 1. Introduction. Why water is essential for life? It is well known that liquid water has played the essential and undeniable role in the emergence, development, and maintenance of life on Earth. Two thirds of the Earth's surface is covered by water, however fresh water is most valuable as a resource for animals and plants. Thus, sustain- ability of our planet's fresh water reserves is an important issue as population numbers increase. Water accounts for 75% of human body mass and is the major constituent of organism fluids. All these facts indicate that water is one of the most important elements for life on Earth. -
Extraterrestrial Hydrogeology
Extraterrestrial hydrogeology Victor R. Baker · James M. Dohm · Alberto G. Fairn · Ty P. A. Ferr · Justin C. Ferris · Hideaki Miyamoto · Dirk Schulze-Makuch Abstract Subsurface water processes are common for conditions delivered water to the northern plains. Water planetary bodies in the solar system and are highly was also cycled to the South Polar Region during changes probable for exoplanets (planets outside the solar system). in climate induced by endogenic activity and/or by For many solar system objects, the subsurface water exists changes in Mars’ orbital parameters. Venus very likely as ice. For Earth and Mars, subsurface saturated zones had a warm hydrosphere for hundreds of millions of have occurred throughout their planetary histories. Earth years, before the development of its current extremely hot is mostly clement with the recharge of most groundwater atmosphere and surface. Subsequently, Venus lost its reservoirs from ample precipitation during transient ice- hydrosphere as solar luminosity increased and a run-away and hot-house conditions, as recorded through the geo- moist greenhouse took effect. Subsurface oceans of water logic and fossilized records. On the other hand, Mars is or ammonia-water composition, induced by tidal forces mostly in an ice-house stage, which is interrupted by and radiogenic heating, probably occur on the larger endogenic-driven activity. This activity catastrophically satellites Europa, Ganymede, Callisto, Titan, and Triton. drives short-lived hydrological cycling and associated Tidal forces operating between some of the small bodies climatic perturbations. Regional aquifers in the Martian of the outer solar system could also promote the fusion of highlands that developed during past, more Earth-like ice and the stability of inner liquid-water oceans. -
Properties of Deuterium-Labeled Hydrates1 *This Lab Was Taken from a Lab Offered at Purdue University
Chem242. Int. Inorg. Chem. UMass-Amherst Properties of Deuterium-Labeled Hydrates1 *This lab was taken from a lab offered at Purdue University Introduction An isotopically labeled compound contains an isotope of one or more of its component elements in higher abundance than is found in nature. Such compounds play an important role in the structural characterization of substances as well as in various mechanistic studies because the presence or location of specific types of atoms can be determined. For example, deuterium (D, the isotope of hydrogen with one proton, one neutron, and an atomic mass of 2) was used in an investigation of the mechanism of the hydrogenation of ethylene, H2C=CH2, to form ethane, CH3CH3, according to the equation: C2H4+H2 → C2H6 This reaction was shown to be more complex than previously thought. When the reaction was run with C2H4 and D2, the product of the reaction was not C2H4D2, as expected, but was a mixture of products: all seven members of the series C2HnD6-n were observed. The mixture of products could be characterized by mass spectrometry because they range in mass from 30 to 36, a consequence of the difference in mass between a normal hydrogen atom and a deuterium atom. Isotopes are also useful in assigning infrared spectra. Changing the mass of a substituent atom by replacing it with a heavier isotope shifts the stretching frequency for normal modes involving that isotope, and thus the infrared bands. Alternatively, the location, of an isotope can sometimes be followed by infrared spectroscopy. Isotopes are also useful for investigating reaction mechanisms, as changing the mass of an atom alters the rate at which it reacts. -
Xenon Hydrate As an Analog of Methane Hydrate in Geologic Systems out of Thermodynamic Equilibrium
Xenon Hydrate as an Analog of Methane Hydrate in Geologic Systems Out of Thermodynamic Equilibrium The MIT Faculty has made this article openly available. Please share how this access benefits you. Your story matters. Citation Fu, Xiaojing et al., "Xenon Hydrate as an Analog of Methane Hydrate in Geologic Systems Out of Thermodynamic Equilibrium." Geochemistry, Geophysics, Geosystems 20, 5 (May 2019): 2462-72 doi. 10.1029/2019GC008250 ©2019 Authors As Published https://dx.doi.org/10.1029/2019GC008250 Publisher American Geophysical Union (AGU) Version Final published version Citable link https://hdl.handle.net/1721.1/125592 Terms of Use Article is made available in accordance with the publisher's policy and may be subject to US copyright law. Please refer to the publisher's site for terms of use. RESEARCH ARTICLE Xenon Hydrate as an Analog of Methane 10.1029/2019GC008250 Hydrate in Geologic Systems Out of Key Points: Thermodynamic Equilibrium • We compare xenon and methane hydrates in terms of equilibrium thermodynamics and Xiaojing Fu1 , William F. Waite2 , Luis Cueto-Felgueroso3,4 , and Ruben Juanes4 nonequilibrium kinetics of hydrate growth 1Department of Earth and Planetary Science, University of California, Berkeley, Berkeley, CA, USA, 2U. S. Geological • Xenon hydrate has a wider Survey, Woods Hole, MA, USA, 3Department of Civil Engineering, Technical University of Madrid, Madrid, Spain, nonstoichiometry region than 4 methane hydrate, which leads to a Department of Civil and Environmental Engineering, Massachusetts Institute of Technology, Cambridge, MA, USA thicker hydrate layer at the gas-liquid interface • Hydrate nonstoichiometry coupled Abstract Methane hydrate occurs naturally under pressure and temperature conditions that are not with hydrate formation dynamics straightforward to replicate experimentally. -
The Hydrates Some Ionic Compounds Have a Number of Water Molecules
Special Ionic Compounds – The Hydrates Some ionic compounds have a number of water molecules hanging around them. These special compounds are called HYDRATES. A hydrate name or formula has two parts – the first part is the ionic compound, the second part is the water. We separate the two parts with a giant asterisk ( *) or dot ( ·) CuSO4 * 5 H2O is named in two steps: The ionic compound is named the regular way, name the cation, name the anion. Next use your molecular prefixes (mono, di, tri etc.) in front of the word hydrate to denote the number of water molecules. Answer: copper (II) sulfate pentahydrate Coming up with the formula for magnesium sulfate heptahydrate is also done in two steps: First come up with the formula for the ionic compound the usual way, by balancing the charges on the ions. Check the prefix to find out how many water (H2O) molecules are with the ionic compound. 2+ 2- Mg with SO4 is MgSO4 and heptahydrate means seven water. Answer: MgSO4 · 7H2O Nomenclature of Acids Acids are hydrogen compounds that are dissolved in water. Technically they are molecular, but unlike molecular compounds they will conduct electricity in water. The formula of an acid is always followed by (aq) which means in aqueous solution, in other words, dissolved in water. Anything dissolved in water has (aq) after its formula. All acids will have it, but just because there is (aq) does NOT mean it is an acid. It is only an acid if it is a hydrogen compound in water. Hydrogen compounds are molecular but they don’t use prefixes and we use the hydrogen to balance out charges with an anion. -
Ethylene Glycol Regeneration Plan: a Systematic Approach to Troubleshoot the Common Problems
21 Journal of Chemical Engineering, IEB Vol. ChE. 27, No. 1, June 2012 Ethylene Glycol Regeneration Plan: A Systematic Approach to Troubleshoot the Common Problems Md. Emdadul Haque Jalalabad Gas Plant, Chevron Bangladesh Abstract Mono Ethylene Glycol (MEG) is used primarily at low-temperature processing plant for extracting natural gas liquids. Typically a physical process plant comprises with gas dehydration system which allows for physical separation of water saturated gas by simple dew point depression and water condensation brought about by chilling from cross exchange with propane refrigerant. The resultant wet gas is prevented from freezing by injection of liquid desiccants to inhibit hydrate formation. The resulting dehydrated gas stream will have a dew point preciously equal to the saturated water volume of the gas at its coolest temperature. Mono Ethylene Glycol has been chosen as hydrate inhibitor because of its low volatility, low toxicity, low flammability, good thermodynamic behavior, and simple proven technology requirement and availability. But it has two common characteristic problems in regeneration plant that is fouling of equipment by iron carbonate, Ca+2/Mg+2 salt deposits and cross contamination of MEG and condensate contamination. MEG in condensate causes condensate specification problems, fouling of condensate stabilization equipment and contamination of wastewater streams. Condensate in MEG causes stripping effect due to condensate vaporization, lower operating temperature, higher MEG purities, and contamination of wastewater streams from MEG Regeneration system and burping of column due to condensate buildup. Another common problem is glycol losses due to carryover with dehydrated gas and which finally accumulates in pipelines and causes corrosion. -
Compound Stoichiometry Unit 5 What Is Stoichiometry?
Compound Stoichiometry Unit 5 What is Stoichiometry? Compound vs Reaction Stoichiometry Molecular Mass Hydrate problems Moles and Avagadro’s Number Percentage Composition Problems Empirical Formula Problems Molecular Formula Problems Hydrates again Stoichiometry Stoichiometry: Element measurement Stoichiometry problems involve calculations (you will need a calculator for this) 2 types of Stoichiometry Problems Compound Stoichiometry Reaction Stoichiometry Types of Compound Stoichiometry Problems Formula/ Molecular Mass Problems Mole Problems Avogadro’s Number Problems Percentage Composition Problems Empirical Formula Problems Molecular Formula Problems Formula/Molecular Mass How to find Formula/Molecular Mass of a compound: write a correct formula for the compound Round the average atomic mass (on periodic table) -round to the nearest whole number- Multiply the Rounded atomic mass of each element but the number of atoms of that element present (usually subscripted) Add the total rounded atomic masses of all elements in the compound and label it with amu: Atomic Mass Units Ex 5-1: Formula/Molecular Mass Problems Find the formula mass of Calcium Phosphate: Ca3(PO4)2 Element # * AMU AMU Ca 3 * 40 = 120 P 2 * 31 = 62 O 8 * 16 = 128 Total AMU: 310 amu Ex 5-2: Find the formula mass of ammonium sulfate: (NH4)2SO4 Ex 5-3: Find the Molecular Mass of dichloride heptoxide: Hydrates Hydrates are ionic compounds which trap water molecules in their crystal structures Need to know how to name and recognize hydrates Formula: Ionic Compound * # H20 Naming: