Effects of Rhythmic Context on Time Perception in Individuals with Parkinson Disease
Total Page:16
File Type:pdf, Size:1020Kb
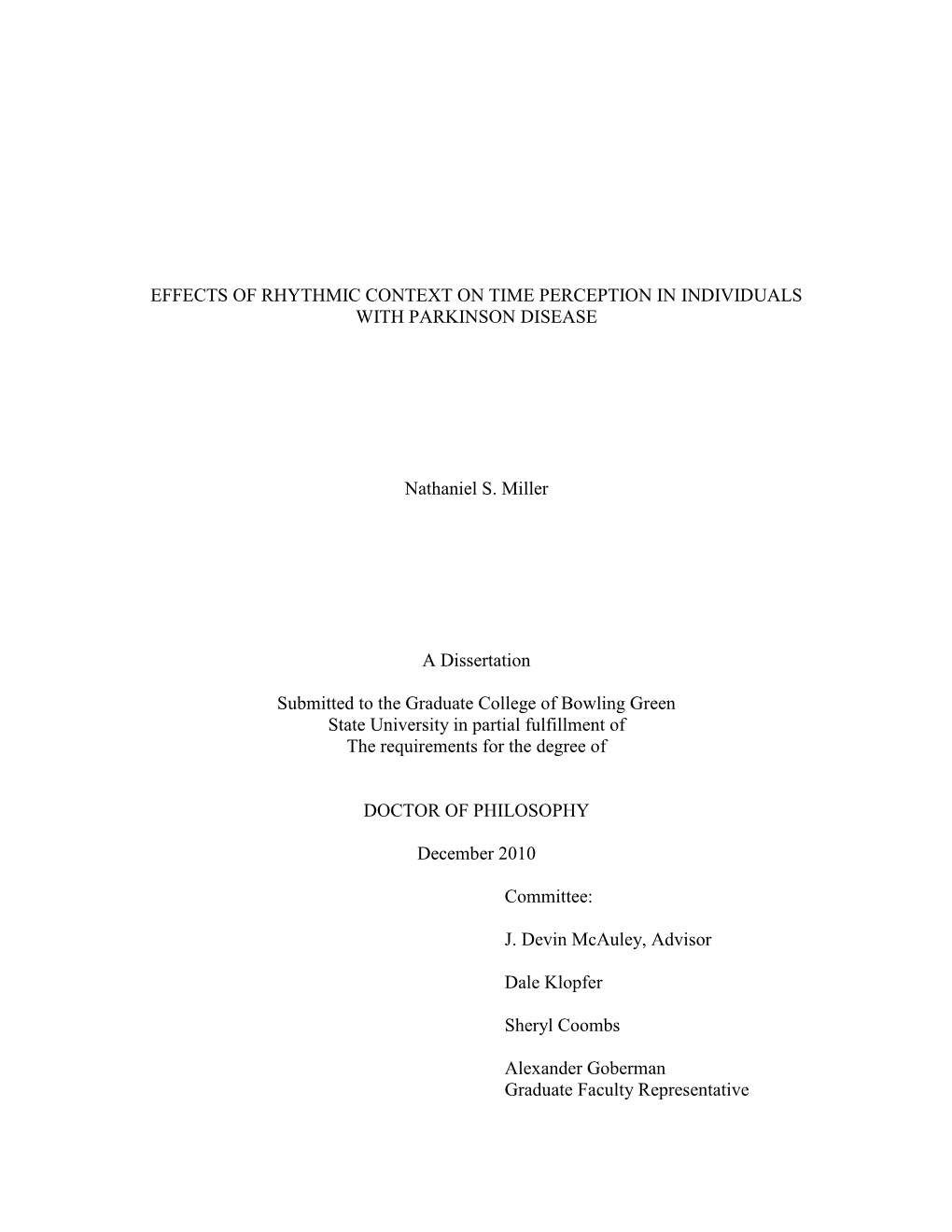
Load more
Recommended publications
-
Severe Organophosphate Poisoning with Delayed Cholinergic Crisis, Intermediate Syndrome and Organophosphate Induced Delayed Polyneuropathy on Succession
Organophosphate Poisoning… Aklilu A 203 CASE REPORT SEVERE ORGANOPHOSPHATE POISONING WITH DELAYED CHOLINERGIC CRISIS, INTERMEDIATE SYNDROME AND ORGANOPHOSPHATE INDUCED DELAYED POLYNEUROPATHY ON SUCCESSION Aklilu Azazh ABSTRACT Organophosphate compounds are the organic derivatives of Phosphorous containing acids and their effect on neuromuscular junction and Autonomic Synapses is clinically important. After exposure these agents cause acute and sub acute manifestations depending on the type and severity of the agents like Acute Cholinergic Manifestations, Intermediate Syndrome with Nicotinic features and Delayed Central Nervous System Complications. The patient reported here had severe Organophosphate Poisoning with various rare complications on a succession. This is the first report of Organophosphates Poisoning complicated by Intermediate Syndrome and Organophosphate Induced Delayed Polyneuropathy in Ethiopia and it is reported to increase awareness of health care workers on these rare complications of a common problem. INTRODUCTION phosphorylated by the Phosphate end of Organophosphates; then the net result is Organophosphate compounds are the organic accumulation of excessive Acetyl Chlorine with derivatives of Phosphorous containing acids and resultant effect on Muscarinic, Nicotinic and their effect on Neuromuscular Junction and central nervous system (Figure 2). Autonomic synapses is clinically important. In the Neuromuscular Junction Acetylcholine is released Following classical OP poisoning, three well when a nerve impulse reaches -
Protocol for a Randomised Controlled Trial: Efficacy of Donepezil Against
BMJ Open: first published as 10.1136/bmjopen-2013-003533 on 25 September 2013. Downloaded from Open Access Protocol Protocol for a randomised controlled trial: efficacy of donepezil against psychosis in Parkinson’s disease (EDAP) Hideyuki Sawada, Tomoko Oeda To cite: Sawada H, Oeda T. ABSTRACT ARTICLE SUMMARY Protocol for a randomised Introduction: Psychosis, including hallucinations and controlled trial: efficacy of delusions, is one of the important non-motor problems donepezil against psychosis Strengths and limitations of this study in patients with Parkinson’s disease (PD) and is in Parkinson’s disease ▪ In previous randomised controlled trials for (EDAP). BMJ Open 2013;3: possibly associated with cholinergic neuronal psychosis the efficacy was investigated in patients e003533. doi:10.1136/ degeneration. The EDAP (Efficacy of Donepezil against who presented with psychosis and the primary bmjopen-2013-003533 Psychosis in PD) study will evaluate the efficacy of endpoint was improvement of psychotic symp- donepezil, a brain acetylcholine esterase inhibitor, for toms. By comparison, this study is designed to prevention of psychosis in PD. ▸ Prepublication history for evaluate the prophylactic effect in patients this paper is available online. Methods and analysis: Psychosis is assessed every without current psychosis. Because psychosis To view these files please 4 weeks using the Parkinson Psychosis Questionnaire may be overlooked and underestimated it is visit the journal online (PPQ) and patients with PD whose PPQ-B score assessed using a questionnaire, Parkinson (http://dx.doi.org/10.1136/ (hallucinations) and PPQ-C score (delusions) have Psychosis Questionnaire (PPQ) every 4 weeks. bmjopen-2013-003533). been zero for 8 weeks before enrolment are ▪ The strength of this study is its prospective randomised to two arms: patients receiving donepezil design using the preset definition of psychosis Received 3 July 2013 hydrochloride or patients receiving placebo. -
The Role of Serotonin in Memory: Interactions with Neurotransmitters and Downstream Signaling
View metadata, citation and similar papers at core.ac.uk brought to you by CORE provided by Bushehr University of Medical Sciences Repository Exp Brain Res (2014) 232:723–738 DOI 10.1007/s00221-013-3818-4 REVIEW The role of serotonin in memory: interactions with neurotransmitters and downstream signaling Mohammad Seyedabadi · Gohar Fakhfouri · Vahid Ramezani · Shahram Ejtemaei Mehr · Reza Rahimian Received: 28 April 2013 / Accepted: 20 December 2013 / Published online: 16 January 2014 © Springer-Verlag Berlin Heidelberg 2014 Abstract Serotonin, or 5-hydroxytryptamine (5-HT), is there has been an alteration in the density of serotonergic found to be involved in many physiological or pathophysi- receptors in aging and Alzheimer’s disease, and serotonin ological processes including cognitive function. Seven dis- modulators are found to alter the process of amyloidogen- tinct receptors (5-HT1–7), each with several subpopulations, esis and exert cognitive-enhancing properties. Here, we dis- have been identified for serotonin, which are different in cuss the serotonin-induced modulation of various systems terms of localization and downstream signaling. Because involved in mnesic function including cholinergic, dopa- of the development of selective agonists and antagonists minergic, GABAergic, glutamatergic transmissions as well for these receptors as well as transgenic animal models as amyloidogenesis and intracellular pathways. of cognitive disorders, our understanding of the role of serotonergic transmission in learning and memory has Keywords Serotonin · Memory · Signaling pathways improved in recent years. A large body of evidence indi- cates the interplay between serotonergic transmission and Abbreviations other neurotransmitters including acetylcholine, dopamine, 2PSDT Two-platform spatial discrimination task γ-aminobutyric acid (GABA) and glutamate, in the neu- 3xTg-AD Triple-transgenic mouse model of Alzheimer’s robiological control of learning and memory. -
Nicotine and Neurotransmitters
Module 2 —Legal Doesn’t Mean Harmless Overview Overview Summary This module focuses on how two drugs, nicotine and alcohol, change the functioning of the brain and body. Both drugs are widely used in the community, and for adults, using them is legal. Nonetheless, both alcohol and nicotine can have a strong impact on the functioning of the brain. Each can cause a number of negative effects on the body and brain, ranging from mild symptoms to addiction. The goal of this module is to help students understand that, although nicotine and alcohol are legal for adults, they are not harmless substances. Students will learn about how nicotine and alcohol change or disrupt the process of neurotransmission. Students will explore information on the short- and long- term effects of these two drugs, and also learn why these drugs are illegal for children and teens. Through the media, students are exposed to a great deal of information about alcohol and tobacco, much of which is misleading or scientifically inaccurate. This module will provide information on what researchers have learned about how nicotine and alcohol change the brain, and the resulting implications for safety and health. Learning Objectives At the end of this module: • Students can explain how nicotine disrupts neurotransmission. • Students can explain how alcohol use may harm the brain and the body. • Students understand how alcohol can intensify the effect of other drugs. • Students can define addiction and understand its basis in the brain. • Students draw conclusions about why our society regulates the use of nicotine and alcohol for young people. -
Neurochemical Mechanisms Underlying Alcohol Withdrawal
Neurochemical Mechanisms Underlying Alcohol Withdrawal John Littleton, MD, Ph.D. More than 50 years ago, C.K. Himmelsbach first suggested that physiological mechanisms responsible for maintaining a stable state of equilibrium (i.e., homeostasis) in the patient’s body and brain are responsible for drug tolerance and the drug withdrawal syndrome. In the latter case, he suggested that the absence of the drug leaves these same homeostatic mechanisms exposed, leading to the withdrawal syndrome. This theory provides the framework for a majority of neurochemical investigations of the adaptations that occur in alcohol dependence and how these adaptations may precipitate withdrawal. This article examines the Himmelsbach theory and its application to alcohol withdrawal; reviews the animal models being used to study withdrawal; and looks at the postulated neuroadaptations in three systems—the gamma-aminobutyric acid (GABA) neurotransmitter system, the glutamate neurotransmitter system, and the calcium channel system that regulates various processes inside neurons. The role of these neuroadaptations in withdrawal and the clinical implications of this research also are considered. KEY WORDS: AOD withdrawal syndrome; neurochemistry; biochemical mechanism; AOD tolerance; brain; homeostasis; biological AOD dependence; biological AOD use; disorder theory; biological adaptation; animal model; GABA receptors; glutamate receptors; calcium channel; proteins; detoxification; brain damage; disease severity; AODD (alcohol and other drug dependence) relapse; literature review uring the past 25 years research- science models used to study with- of the reasons why advances in basic ers have made rapid progress drawal neurochemistry as well as a research have not yet been translated Din understanding the chemi- reluctance on the part of clinicians to into therapeutic gains and suggests cal activities that occur in the nervous consider new treatments. -
Two Types of Muscarinic Response to Acetylcholine in Mammalian Cortical Neurons (Clngulate/M Current/Cholinergic/Pirenzepine) DAVID A
Proc. Nail. Acad. Sci. USA Vol. 82, pp. 6344-6348, September 1985 Neurobiology Two types of muscarinic response to acetylcholine in mammalian cortical neurons (clngulate/M current/cholinergic/pirenzepine) DAVID A. MCCORMICK AND DAVID A. PRINCE Department of Neurology, Room C338, Stanford University School of Medicine, Stanford, CA 94305 Communicated by Richard F. Thompson, May 22, 1985 ABSTRACT Applications of acetylcholine (AcCho) to py- The cerebral cortex contains nicotinic as well as several ramidal cells of guinea pig cingulate cortical slices maintained subtypes of muscarinic AcCho receptors (20-23). Previous in vitro result in a short latency inhibition, followed by a reports suggest that cholinergic slow excitation is mediated prolonged increase in excitability. Cholinergic inhibition is by receptors possessing muscarinic characteristics, while mediated through the rapid excitation of interneurons that cholinergic inhibition may be due to activation of receptors utilize the inhibitory neurotransmitter y-aminobutyric acid that have both nicotinic and muscarinic properties (5, 24). (GABA). This rapid excitation of interneurons is. associated The recent characterization of receptor antagonists (e.g., with a membrane depolarization and a decrease in neuronal pirenzepine) and agonists (e.g., pilocarpine) that are relative- input resistance. In contrast, AcCho-induced excitation of ly specific for subtypes ofmuscarinic receptors (21, 22) raises pyramidal cells is due to a direct action that produces a the question of whether different types of cholinergic re- voltage-dependent increase in input resistance. In the experi- sponses demonstrated physiologically within the central ments reported here, we investigated the possibility that these nervous system might be due to activation of different two responses are mediated by different subclasses of cholin- subclasses of muscarinic receptors, as appears to be the case ergic receptors. -
Drug Class Review Ophthalmic Cholinergic Agonists
Drug Class Review Ophthalmic Cholinergic Agonists 52:40.20 Miotics Acetylcholine (Miochol-E) Carbachol (Isopto Carbachol; Miostat) Pilocarpine (Isopto Carpine; Pilopine HS) Final Report November 2015 Review prepared by: Melissa Archer, PharmD, Clinical Pharmacist Carin Steinvoort, PharmD, Clinical Pharmacist Gary Oderda, PharmD, MPH, Professor University of Utah College of Pharmacy Copyright © 2015 by University of Utah College of Pharmacy Salt Lake City, Utah. All rights reserved. Table of Contents Executive Summary ......................................................................................................................... 3 Introduction .................................................................................................................................... 4 Table 1. Glaucoma Therapies ................................................................................................. 5 Table 2. Summary of Agents .................................................................................................. 6 Disease Overview ........................................................................................................................ 8 Table 3. Summary of Current Glaucoma Clinical Practice Guidelines ................................... 9 Pharmacology ............................................................................................................................... 10 Methods ....................................................................................................................................... -
Therapeutic Effect of Agmatine on Neurological Disease: Focus on Ion Channels and Receptors
Neurochemical Research (2019) 44:735–750 https://doi.org/10.1007/s11064-018-02712-1 REVIEW PAPER Therapeutic Effect of Agmatine on Neurological Disease: Focus on Ion Channels and Receptors Sumit Barua1 · Jong Youl Kim1 · Jae Young Kim1 · Jae Hwan Kim4 · Jong Eun Lee1,2,3 Received: 15 October 2018 / Revised: 19 December 2018 / Accepted: 24 December 2018 / Published online: 4 January 2019 © Springer Science+Business Media, LLC, part of Springer Nature 2019 Abstract The central nervous system (CNS) is the most injury-prone part of the mammalian body. Any acute or chronic, central or peripheral neurological disorder is related to abnormal biochemical and electrical signals in the brain cells. As a result, ion channels and receptors that are abundant in the nervous system and control the electrical and biochemical environment of the CNS play a vital role in neurological disease. The N-methyl-D-aspartate receptor, 2-amino-3-(5-methyl-3-oxo-1,2-oxazol-4-yl) propanoic acid receptor, kainate receptor, acetylcholine receptor, serotonin receptor, α2-adrenoreceptor, and acid-sensing ion channels are among the major channels and receptors known to be key components of pathophysiological events in the CNS. The primary amine agmatine, a neuromodulator synthesized in the brain by decarboxylation of L-arginine, can regu- late ion channel cascades and receptors that are related to the major CNS disorders. In our previous studies, we established that agmatine was related to the regulation of cell differentiation, nitric oxide synthesis, and murine brain endothelial cell migration, relief of chronic pain, cerebral edema, and apoptotic cell death in experimental CNS disorders. -
Agmatine and Agmatine Analogs in the Treatment of Epilepsy, Seizure, and Electroconvulsive Disorders Peter A
University of Kentucky UKnowledge Pharmaceutical Sciences Faculty Patents Pharmaceutical Sciences 10-19-2010 Agmatine and Agmatine Analogs in the Treatment of Epilepsy, Seizure, and Electroconvulsive Disorders Peter A. Crooks University of Kentucky, [email protected] Aimee K. Bence David R. Worthen Right click to open a feedback form in a new tab to let us know how this document benefits oy u. Follow this and additional works at: https://uknowledge.uky.edu/ps_patents Part of the Pharmacy and Pharmaceutical Sciences Commons Recommended Citation Crooks, Peter A.; Bence, Aimee K.; and Worthen, David R., "Agmatine and Agmatine Analogs in the Treatment of Epilepsy, Seizure, and Electroconvulsive Disorders" (2010). Pharmaceutical Sciences Faculty Patents. 47. https://uknowledge.uky.edu/ps_patents/47 This Patent is brought to you for free and open access by the Pharmaceutical Sciences at UKnowledge. It has been accepted for inclusion in Pharmaceutical Sciences Faculty Patents by an authorized administrator of UKnowledge. For more information, please contact [email protected]. US007816407B2 (12) United States Patent (10) Patent N0.: US 7,816,407 B2 Crooks et al. (45) Date of Patent: Oct. 19, 2010 (54) AGMATINE AND AGMATINE ANALOGS IN The Merck Index, Merck Research Laboratories Division of Merck & THE TREATMENT OF EPILEPSY, SEIZURE, Co., Inc. 1996, p. 35. AND ELECTROCONVULSIVE DISORDERS James O. McNamara, “Drugs Effective in Therapy of the Epilepsies”, Goodman & Gilman’s The Pharmacological Basis of Therapeutics, (75) Inventors: Peter A. Crooks, Lexington, KY (US); Ninth Edition, Chapter 20, pp. 461-486, 1996. Aimee K. Bence, Lexington, KY (US); I. Tayfun Uzbay et al., “Effects of agmatine on ethanol Withdrawal David R. -
Redalyc.Neurobiological Alterations in Alcohol Addiction: a Review
Adicciones ISSN: 0214-4840 [email protected] Sociedad Científica Española de Estudios sobre el Alcohol, el Alcoholismo y las otras Toxicomanías España Erdozain, Amaia M.; Callado, Luis F. Neurobiological alterations in alcohol addiction: a review Adicciones, vol. 26, núm. 4, octubre-diciembre, 2014, pp. 360-370 Sociedad Científica Española de Estudios sobre el Alcohol, el Alcoholismo y las otras Toxicomanías Palma de Mallorca, España Available in: http://www.redalyc.org/articulo.oa?id=289132934009 How to cite Complete issue Scientific Information System More information about this article Network of Scientific Journals from Latin America, the Caribbean, Spain and Portugal Journal's homepage in redalyc.org Non-profit academic project, developed under the open access initiative revisión adicciones vol. 26, nº 3 · 2014 Neurobiological alterations in alcohol addiction: a review Alteraciones neurobiológicas en el alcoholismo: revisión Amaia M. Erdozain*,*** and Luis F. Callado*,** *Department of Pharmacology, University of the Basque Country UPV/EHU, Leioa, Bizkaia, Spain and Centro de Investigación Biomédica en Red de Salud Mental (CIBERSAM), Spain. **Biocruces Health Research Institute, Bizkaia, Spain. ***Neuroscience Paris Seine, Université Pierre et Marie Curie, Paris, France Resumen Abstract Todavía se desconoce el mecanismo exacto mediante el cual el etanol The exact mechanism by which ethanol exerts its effects on the brain produce sus efectos en el cerebro. Sin embargo, hoy en día se sabe is still unknown. However, nowadays it is well known that ethanol que el etanol interactúa con proteínas específicas de la membrana interacts with specific neuronal membrane proteins involved in neuronal, implicadas en la transmisión de señales, produciendo así signal transmission, resulting in changes in neural activity. -
The Efficacy and Safety of Six-Weeks of Pre-Workout Supplementation in Resistance Trained Rats
View metadata, citation and similar papers at core.ac.uk brought to you by CORE provided by College of William & Mary: W&M Publish W&M ScholarWorks Undergraduate Honors Theses Theses, Dissertations, & Master Projects 4-2017 The Efficacy and Safety of Six-Weeks of Pre-Workout Supplementation in Resistance Trained Rats Justin P. Canakis College of William and Mary Follow this and additional works at: https://scholarworks.wm.edu/honorstheses Part of the Animal Sciences Commons, Exercise Science Commons, Laboratory and Basic Science Research Commons, and the Other Nutrition Commons Recommended Citation Canakis, Justin P., "The Efficacy and Safety of Six-Weeks of Pre-Workout Supplementation in Resistance Trained Rats" (2017). Undergraduate Honors Theses. Paper 1128. https://scholarworks.wm.edu/honorstheses/1128 This Honors Thesis is brought to you for free and open access by the Theses, Dissertations, & Master Projects at W&M ScholarWorks. It has been accepted for inclusion in Undergraduate Honors Theses by an authorized administrator of W&M ScholarWorks. For more information, please contact [email protected]. 1 2 Title Page……………………………………………………………………………………...…..1 Abstract…………………………………………………………………………………………....5 Acknowledgement……………………………………………………………………….………..6 Background.………………………………………………………………………..……………...7 DSEHA and its Effect on the VMS Industry………………...……………………………7 History of Adverse Side Effects from Pre-Workout Supplements ………….……………8 Ingredient Analysis ………………………………………..……………….……………..……....9 2.5g Beta-Alanine…………………………..……………………………………………..9 -
[3H]Acetylcholine to Muscarinic Cholinergic Receptors’
0270.6474/85/0506-1577$02.00/O The Journal of Neuroscience CopyrIght 0 Smety for Neurosmnce Vol. 5, No. 6, pp. 1577-1582 Prrnted rn U S.A. June 1985 High-affinity Binding of [3H]Acetylcholine to Muscarinic Cholinergic Receptors’ KENNETH J. KELLAR,2 ANDREA M. MARTINO, DONALD P. HALL, Jr., ROCHELLE D. SCHWARTZ,3 AND RICHARD L. TAYLOR Department of Pharmacology, Georgetown University, Schools of Medicine and Dentistry, Washington, DC 20007 Abstract affinities (Birdsall et al., 1978). Evidence for this was obtained using the agonist ligand [3H]oxotremorine-M (Birdsall et al., 1978). High-affinity binding of [3H]acetylcholine to muscarinic Studies of the actions of muscarinic agonists and detailed analy- cholinergic sites in rat CNS and peripheral tissues was meas- ses of binding competition curves between muscarinic agonists and ured in the presence of cytisin, which occupies nicotinic [3H]antagonists have led to the concept of muscarinic receptor cholinergic receptors. The muscarinic sites were character- subtypes (Rattan and Goyal, 1974; Goyal and Rattan, 1978; Birdsall ized with regard to binding kinetics, pharmacology, anatom- et al., 1978). This concept was reinforced by the discovery of the ical distribution, and regulation by guanyl nucleotides. These selective actions and binding properties of the antagonist pirenze- binding sites have characteristics of high-affinity muscarinic pine (Hammer et al., 1980; Hammer and Giachetti, 1982; Watson et cholinergic receptors with a Kd of approximately 30 nM. Most al., 1983; Luthin and Wolfe, 1984). An evolving classification scheme of the muscarinic agonist and antagonist drugs tested have for these muscarinic receptors divides them into M-l and M-2 high affinity for the [3H]acetylcholine binding site, but piren- subtypes (Goyal and Rattan, 1978; for reviews, see Hirschowitz et zepine, an antagonist which is selective for M-l receptors, al., 1984).