Next Generation Electrical Energy Storage
Total Page:16
File Type:pdf, Size:1020Kb
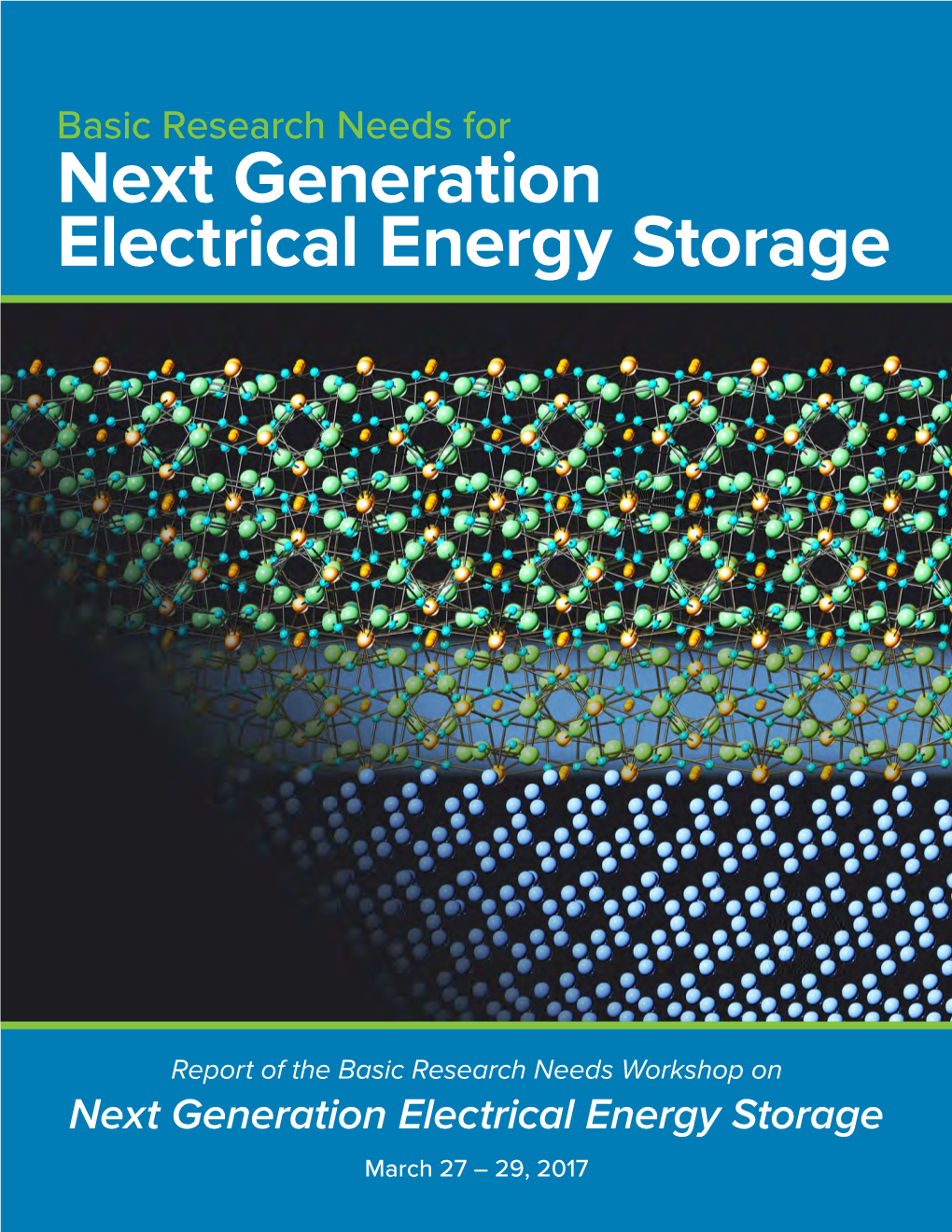
Load more
Recommended publications
-
A Review of Functional Separators for Lithium Metal Battery Applications
materials Review A Review of Functional Separators for Lithium Metal Battery Applications Jooyoung Jang y, Jiwoong Oh y, Hyebin Jeong y, Woosuk Kang y and Changshin Jo * School of Chemical Engineering & Materials Science, Chung-Ang University (CAU), 84, Heukseok-ro, Dongjakgu, Seoul 06974, Korea; [email protected] (J.J.); [email protected] (J.O.); [email protected] (H.J.); [email protected] (W.K.) * Correspondence: [email protected]; Tel.: +82-2-820-5477 These authors contributed equally to this work. y Received: 31 August 2020; Accepted: 12 October 2020; Published: 16 October 2020 Abstract: Lithium metal batteries are considered “rough diamonds” in electrochemical energy storage systems. Li-metal anodes have the versatile advantages of high theoretical capacity, low density, and low reaction potential, making them feasible candidates for next-generation battery applications. However, unsolved problems, such as dendritic growths, high reactivity of Li-metal, low Coulombic efficiency, and safety hazards, still exist and hamper the improvement of cell performance and reliability. The use of functional separators is one of the technologies that can contribute to solving these problems. Recently, functional separators have been actively studied and developed. In this paper, we summarize trends in the research on separators and predict future prospects. Keywords: lithium metal battery; separator; next-generation batteries 1. Introduction Rechargeable batteries are chemical energy storage systems that interconvert chemical energy and electrical energy through the redox reactions of cathode and anode materials. Fossil fuel-based energy cycles are being replaced by renewable energy cycles at a high pace. In this regard, energy storage devices have proven to be essential in fulfilling the global demands of sustainable energy supply and solving problems such as oil depletion and environmental pollution [1–5]. -
A Highly Controllable Electrochemical Anodization Process to Fabricate
Lin et al. Nanoscale Research Letters (2015) 10:495 DOI 10.1186/s11671-015-1202-y NANO EXPRESS Open Access A Highly Controllable Electrochemical Anodization Process to Fabricate Porous Anodic Aluminum Oxide Membranes Yuanjing Lin1, Qingfeng Lin1, Xue Liu1, Yuan Gao1, Jin He2, Wenli Wang3,4* and Zhiyong Fan1* Abstract Due to the broad applications of porous alumina nanostructures, research on fabrication of anodized aluminum oxide (AAO) with nanoporous structure has triggered enormous attention. While fabrication of highly ordered nanoporous AAO with tunable geometric features has been widely reported, it is known that its growth rate can be easily affected by the fluctuation of process conditions such as acid concentration and temperature during electrochemical anodization process. To fabricate AAO with various geometric parameters, particularly, to realize precise control over pore depth for scientific research and commercial applications, a controllable fabrication process is essential. In this work, we revealed a linear correlation between the integrated electric charge flow throughout the circuit in the stable anodization process and the growth thickness of AAO membranes. With this understanding, we developed a facile approach to precisely control the growth process of the membranes. It was found that this approach is applicable in a large voltage range, and it may be extended to anodization of other metal materials such as Ti as well. Keywords: Anodic aluminum oxide, Nanoporous structure, Integrated charge density, Controllable electrochemical anodization Background aluminum oxide (AAO), have wide nanoengineering ap- Metal anodization has been broadly used in industry as plications that have attracted enormous attention. For a surface treatment technique to render materials with example, AAO membranes have been used as templates resistance against uncontrolled oxidation, abrasion, and to directly assemble semiconductor nanowires and corrosion. -
The Role and Utilization of Pseudocapacitance for Energy Storage by Supercapacitors
University of Calgary PRISM: University of Calgary's Digital Repository Science Science Research & Publications 1997 The role and utilization of pseudocapacitance for energy storage by supercapacitors Birss, Viola I.; Conway, B. E.; Wojtowicz, J. Elsevier Conway. B. E., Birss, V. I. and Wojtowicz, J. (1997). "The role and utilization of pseudocapacitance for energy storage by supercapacitors." Journal of Power Sources, Vol. 66, Nos. 1-2: 1-14. http://hdl.handle.net/1880/44956 journal article Downloaded from PRISM: https://prism.ucalgary.ca POWER ELSEVIER Journal of Power Sources 66 (1997) 1-14 The role and utilization of pseudocapacitance for energy storage by supercapacitors B.E. Conway, V. Birss l, J. Wojtowicz 2 Chemistry Department, University of Ottawa, Ottawa, Ont., KIN 6N5, Canada Abstract The principle of utilizing the non-Faradaic double-layer capacitance of electrode interfaces as a means of storinc electrical energy was suggested and utilized in technologies initiated some 37 years ago. However, only over the last ten years has major interest been manifested in commercial development of this possibility in so-called 'supercapacitors' or 'ultracapacitors' based on the large double-layer capacitance achievable at high-area, carbon powder electrodes. In parallel with the utilization of double-layer capacitance is the possibility of use of the large pseudocapacitance that is associated with e.g. electrosorption of H or metal adatoms (underpotential deposition) and especially some redox processes. Such pseudocapacitance arises when, -
Call for Papers | 2022 MRS Spring Meeting
Symposium CH01: Frontiers of In Situ Materials Characterization—From New Instrumentation and Method to Imaging Aided Materials Design Advancement in synchrotron X-ray techniques, microscopy and spectroscopy has extended the characterization capability to study the structure, phonon, spin, and electromagnetic field of materials with improved temporal and spatial resolution. This symposium will cover recent advances of in situ imaging techniques and highlight progress in materials design, synthesis, and engineering in catalysts and devices aided by insights gained from the state-of-the-art real-time materials characterization. This program will bring together works with an emphasis on developing and applying new methods in X-ray or electron diffraction, scanning probe microscopy, and other techniques to in situ studies of the dynamics in materials, such as the structural and chemical evolution of energy materials and catalysts, and the electronic structure of semiconductor and functional oxides. Additionally, this symposium will focus on works in designing, synthesizing new materials and optimizing materials properties by utilizing the insights on mechanisms of materials processes at different length or time scales revealed by in situ techniques. Emerging big data analysis approaches and method development presenting opportunities to aid materials design are welcomed. Discussion on experimental strategies, data analysis, and conceptual works showcasing how new in situ tools can probe exotic and critical processes in materials, such as charge and heat transfer, bonding, transport of molecule and ions, are encouraged. The symposium will identify new directions of in situ research, facilitate the application of new techniques to in situ liquid and gas phase microscopy and spectroscopy, and bridge mechanistic study with practical synthesis and engineering for materials with a broad range of applications. -
Comparison of Lithium-Ion Anode Materials Using an Experimentally Verified Physics-Based Electrochemical Model
energies Article Comparison of Lithium-Ion Anode Materials Using an Experimentally Verified Physics-Based Electrochemical Model Rujian Fu 1, Xuan Zhou 2,*, Hengbin Fan 2, Douglas Blaisdell 2, Ajay Jagadale 2, Xi Zhang 3 ID and Rui Xiong 4 ID 1 Independent Researcher, Novi, MI 48377, USA; [email protected] 2 Department of Electrical and Computer Engineering, Kettering University, Flint, MI 48504, USA; [email protected] (H.F.); [email protected] (D.B.); [email protected] (A.J.) 3 School of Mechanical Engineering, Shanghai Jiao Tong University, 800 Dongchuan Rd., Shanghai 200240, China; [email protected] 4 National Engineering Laboratory for Electric Vehicles and Collaborative Innovation Center of Electric Vehicles in Beijing, Beijing Institute of Technology, Beijing 100081, China; [email protected] * Correspondence: [email protected]; Tel.: +1-850-762-9500 Received: 17 November 2017; Accepted: 12 December 2017; Published: 19 December 2017 Abstract: Researchers are in search of parameters inside Li-ion batteries that can be utilized to control their external behavior. Physics-based electrochemical model could bridge the gap between Li+ transportation and distribution inside battery and battery performance outside. In this paper, two commercially available Li-ion anode materials: graphite and Lithium titanate (Li4Ti5O12 or LTO) were selected and a physics-based electrochemical model was developed based on half-cell assembly and testing. It is found that LTO has a smaller diffusion coefficient (Ds) than graphite, which causes a larger overpotential, leading to a smaller capacity utilization and, correspondingly, a shorter duration of constant current charge or discharge. However, in large current applications, LTO performs better than graphite because its effective particle radius decreases with increasing current, leading to enhanced diffusion. -
Effect of Radiation on the Morphology of Lithium-Ion Battery Cathodes
Effect of Radiation on the Morphology of Lithium-ion Battery Cathodes Thesis Presented in Partial Fulfillment of the Requirements for the Degree Master of Science in the Graduate School of The Ohio State University By Dandan He, B.S. Graduate Program in Nuclear Engineering The Ohio State University 2014 Thesis Committee: Lei Cao, Advisor Tunc Aldemir Mingzhai Sun Copyright by Dandan He 2014 Abstract The Lithium ion (Li-ion) battery is widely used as power source for consumer electronic devices due to its high energy density, large specific capacity. Recently, application of the Li-ion battery has been extended to aerospace, in which the outer space’s radiation environment has more stringent requirements for the battery performance. This type of battery also provides power for critical modern rescue, sampling equipment in nuclear environment, such as the robots deployed in the aftermath of Fukushima nuclear accident. As one of the most important components of these emergency response robots, the stability of the Li-ion battery under radiation is of crucial importance. The radiation effects on materials are generally categorized into four types: ionization, atomic displacement, impurity production, and energy release. To our knowledge, there has been no definitive study of such effects on Li-ion batteries and how ionizing radiation affects Li-ion batteries’ structure, strength, deformation, and electrical properties to final failure. ii In this work, the surface morphology of the Li-ion battery cathode before and after neutron and gamma ray radiation were characterized by atomic force microscopy (AFM). Distinct particle coarsening (size increase) of the cathode after irradiation was observed, which may primarily came from the crystal boundary migration driven by internal stress due to irradiation. -
Design and Fabrication of High Power Microbatteries and High Specific Strength Cellular Solids from Bicontinuous Microporous Hierarchical Materials
DESIGN AND FABRICATION OF HIGH POWER MICROBATTERIES AND HIGH SPECIFIC STRENGTH CELLULAR SOLIDS FROM BICONTINUOUS MICROPOROUS HIERARCHICAL MATERIALS BY JAMES HENRY PIKUL DISSERTATION Submitted in partial fulfillment of the requirements for the degree of Doctor of Philosophy in Mechanical Engineering in the Graduate College of the University of Illinois at Urbana-Champaign, 2015 Urbana, Illinois Doctoral Committee: Professor William King, Chair and Director of Research Professor Paul Braun Professor Placid Ferreira Professor John Rogers ABSTRACT An emerging paradigm in engineering design is the development of materials by constructing hierarchical assemblies of simple building blocks into complex architectures that address physics at multiple length scales. These hierarchical materials are increasingly important for the next generation of mechanical, electrical, chemical, and biological technologies. However, fabricating hierarchical materials with nm control over multiple chemistries in a scalable fashion is a challenge yet to be overcome. This dissertation reports the design and fabrication of hierarchical microbattery electrodes that demonstrate unprecedented power density as well as hierarchical cellular solids with controllable modulus and high specific strength. Self-assembly, electrodeposition and microfabrication enable the fabrication of microbatteries with hierarchical electrodes. The three-dimensional bicontinuous interdigitated microelectrode architecture improves power performance by simultaneously reducing ion and electron transport distances through the anode, cathode, and electrolyte. The microbattery power densities are up to 7.4 mW cm-2 m-1, which equals or exceeds that of the best supercapacitors and which is 2000 times higher than that of other microbatteries. A one dimensional electrochemical model of the microbatteries enables the study of physical processes that limit power performance. -
Enhanced Conductivity and Ion Exchange Capacity of Chitosan Membranes Through Modification with Lithium for Lithium Polymer Battery Application
S. N. Asnin, Wahab, D. Permana, L. O. Ahmad, WSEAS TRANSACTIONS on POWER SYSTEMS S. H. Sabarwati, L. O. A. N. Ramadhan Enhanced Conductivity and Ion Exchange Capacity of Chitosan Membranes through Modification with Lithium for Lithium Polymer Battery Application S. N. ASNIN1, WAHAB2, D. PERMANA3, L. O. AHMAD1*, S. H. SABARWATI1, L. O. A. N. RAMADHAN1 1Department of Chemistry, Universitas Halu Oleo, Kendari 2Department of Mining Engineering, Universitas Halu Oleo, Kendari 3 Department of Agriculture, University of Sembilanbelas November, Kolaka INDONESIA *Corresponding author e-mail: [email protected] http://www.uho.ac.id Abstract: - Modification of chitosan membranes using lithium has been developed. The chitosan membranes were prepared from several deacetylation degree of chitosan. The effect of the deacetylation degree towards an ion conductivity properties of the chitosan membranes, effect of concentration of chitosan-lithium membranes and capability of ionic conductivity as an electrolyte polymer for lithium polymer battery application were studied. The membranes were prepared by the solvent evaporation method. Fourier transform-infrared -1 spectroscopy showed a peak at 1573.8 cm correspond to the ionic interaction between lithium salt with NH2 groups from chitosan. The scanning electron microscopy analysis showed high pore density and rigidity of chitosan membrane microstructure, meanwhile chitosan-lithium membranes have clusters which indicated the deposition of lithium nitrate salt at chitosan polymer chains. The water uptake capacity of the chitosan-lithium membranes was higher than that of the chitosan membrane. The tensile strength analysis showed that the chitosan-lithium membranes was more elastic than the chitosan membranes. Chitosan membrane conductivity -2 -1 -1 and ion exchange capacity are 1.70 x 10 S.cm and 2.11 meq.g , while the conductivity after adding lithium -2 -1 -1 salt increased to 8.53 x 10 S.cm and 3.16 meq.g , respectively. -
Technical Summaries with Index
Energy Frontier Research Centers Technical Summaries September 2020 https://science.osti.gov/bes/efrc INTRODUCTION Since its inception in 2009, the U. S. Department of Energy’s Energy Frontier Research Center (EFRC) program has become an important research modality in the Department’s portfolio, enabling high impact research that addresses key scientific challenges for energy technologies. Funded by the Office of Science’s Basic Energy Sciences program, the EFRCs are located across the United States and are led by universities, national laboratories, and private research institutions. These multi-investigator, multi- disciplinary centers bring together world-class teams of researchers, often from multiple institutions, to tackle the toughest scientific challenges preventing advances in energy technologies. In 2009 five-year awards were made to 46 EFRCs, including 16 that were fully funded by the American Recovery and Reinvestment Act (ARRA). An open recompetition of the program in 2014 resulted in four- year awards to 32 centers, 22 of which were renewals of existing EFRCs and 10 of which were new EFRCs. In 2016, DOE added 4 new four-year centers to accelerate the scientific breakthroughs needed to support the Department’s environmental management and nuclear cleanup mission. An open recompetition in 2018 resulted in 42 awards: 11 two-year extensions of existing EFRCs, 9 four-year renewals of existing EFRCs, and 22 four-year awards for new EFRCs. A targeted competition in environmental management, microelectronics, polymer upcycling, and quantum information science in 2020 resulted in 10 awards: 2 two-year extensions of existing EFRCs, 2 four-year renewals of existing EFRCs, and 6 four-year awards for new EFRCs. -
Recent Studies on Supercapacitors with Next-Generation Structures
micromachines Review Recent Studies on Supercapacitors with Next-Generation Structures Juho Sung and Changhwan Shin * Department of Electrical and Computer Engineering, Sungkyunkwan University, Suwon 16419, Korea; [email protected] * Correspondence: [email protected]; Tel.: +82-31-290-7694 Received: 27 November 2020; Accepted: 16 December 2020; Published: 18 December 2020 Abstract: Supercapacitors have shown great potential as a possible solution to the increasing global demand for next-generation energy storage systems. Charge repositioning is based on physical or chemical mechanisms. There are three types of supercapacitors—the electrochemical double layer, the pseudocapacitor, and a hybrid of both. Each type is further subdivided according to the material used. Herein, a detailed overview of the working mechanism as well as a new method for capacitance enhancement are presented. Keywords: supercapacitor; electrical double layer capacitor; EDLC; negative capacitance; energy storage system; pseudocapacitor; micropores; nanopores; ultracapacitor; Helmholtz model 1. Introduction The Fourth Industrial Revolution, which facilitates the emergence of a hyper-connected society, is the basis for data processing between people and things as well as things and things. The essence anchored in a host of electronic devices highlights the energy consumption problem to address the ever-increasing energy demand. However, the challenge confronted (based on expenditure) is proving hard to overcome. Self-powered devices are mostly based on lithium-ion batteries, which were proposed three decades ago. They demonstrate superior properties as compared to energy storage systems (ESSs) based on other materials with the highest energy storage capacity; however, the world no longer refers to them as “perfect batteries”. Moreover, the progress of battery technology would be considered as a stumbling block to the development of electrically powered industry in some parts of the world. -
JES2016-Transport Pseudocaps Galvanostatic.Pdf
Journal of The Electrochemical Society, 163 (2) A229-A243 (2016) A229 Electrochemical Transport Phenomena in Hybrid Pseudocapacitors under Galvanostatic Cycling Anna L. d’Entremont, Henri-Louis Girard, Hainan Wang, and Laurent Pilon∗,z Henry Samueli School of Engineering and Applied Science, Mechanical and Aerospace Engineering Department, University of California, Los Angeles, Los Angeles, California 90095, USA This study aims to provide insights into the electrochemical transport and interfacial phenomena in hybrid pseudocapacitors under galvanostatic cycling. Pseudocapacitors are promising electrical energy storage devices for applications requiring large power density. They also involve complex, coupled, and multiscale physical phenomena that are difficult to probe experimentally. The present study performed detailed numerical simulations for a hybrid pseudocapacitor with planar electrodes and binary, asymmetric electrolyte under various cycling conditions, based on a first-principles continuum model accounting simultaneously for charge storage by electric double layer (EDL) formation and by faradaic reactions with intercalation. Two asymptotic regimes were identified corresponding to (i) dominant faradaic charge storage at low current and low frequency or (ii) dominant EDL charge storage at high current and high frequency. Analytical expressions for the intercalated ion concentration and surface overpotential were derived for both asymptotic regimes. Features of typical experimentally measured cell potential were physically interpreted. These insights could guide the optimization of hybrid pseudocapacitors. © The Author(s) 2015. Published by ECS. This is an open access article distributed under the terms of the Creative Commons Attribution Non-Commercial No Derivatives 4.0 License (CC BY-NC-ND, http://creativecommons.org/licenses/by-nc-nd/4.0/), which permits non-commercial reuse, distribution, and reproduction in any medium, provided the original work is not changed in any way and is properly cited. -
Pseudocapacitive Oxide Materials for High-Rate Electrochemical Energy Storage
Open Archive Toulouse Archive Ouverte (OATAO) OATAO is an open access repository that collects the work of Toulouse researchers and makes it freely available over the web where possible. This is an author-deposited version published in: http://oatao.univ-toulouse.fr/ Eprints ID: 13900 To link to this article : DOI:10.1039/c3ee44164d URL : http://dx.doi.org/10.1039/c3ee44164d To cite this version: Augustyn, Veronica and Simon, Patrice and Dunn, Bruce Pseudocapacitive oxide materials for high-rate electrochemical energy storage. (2014) Energy & Environmental Science, vol. 7 (n° 5). pp. 1597-1614. ISSN 1754-5692 Any correspondence concerning this service should be sent to the repository administrator: [email protected] Pseudocapacitive oxide materials for high-rate electrochemical energy storage Veronica Augustyn,†a Patrice Simonbc and Bruce Dunn*a Electrochemical energy storage technology is based on devices capable of exhibiting high energy density (batteries) or high power density (electrochemical capacitors). There is a growing need, for current and near-future applications, where both high energy and high power densities are required in the same material. Pseudocapacitance, a faradaic process involving surface or near surface redox reactions, offers a means of achieving high energy density at high charge–discharge rates. Here, we focus on the pseudocapacitive properties of transition metal oxides. First, we introduce pseudocapacitance and describe its electrochemical features. Then, we review the most relevant pseudocapacitive materials in DOI: 10.1039/c3ee44164d aqueous and non-aqueous electrolytes. The major challenges for pseudocapacitive materials along with a future outlook are detailed at the end. Broader context The importance of electrical energy storage will continue to grow as markets for consumer electronics and electrication of transportation expand and energy storage systems for renewable energy sources begin to emerge.