Fine Root Dynamics in Afromontane Forest and Adjacent Land Uses in the Northwest Ethiopian Highlands
Total Page:16
File Type:pdf, Size:1020Kb
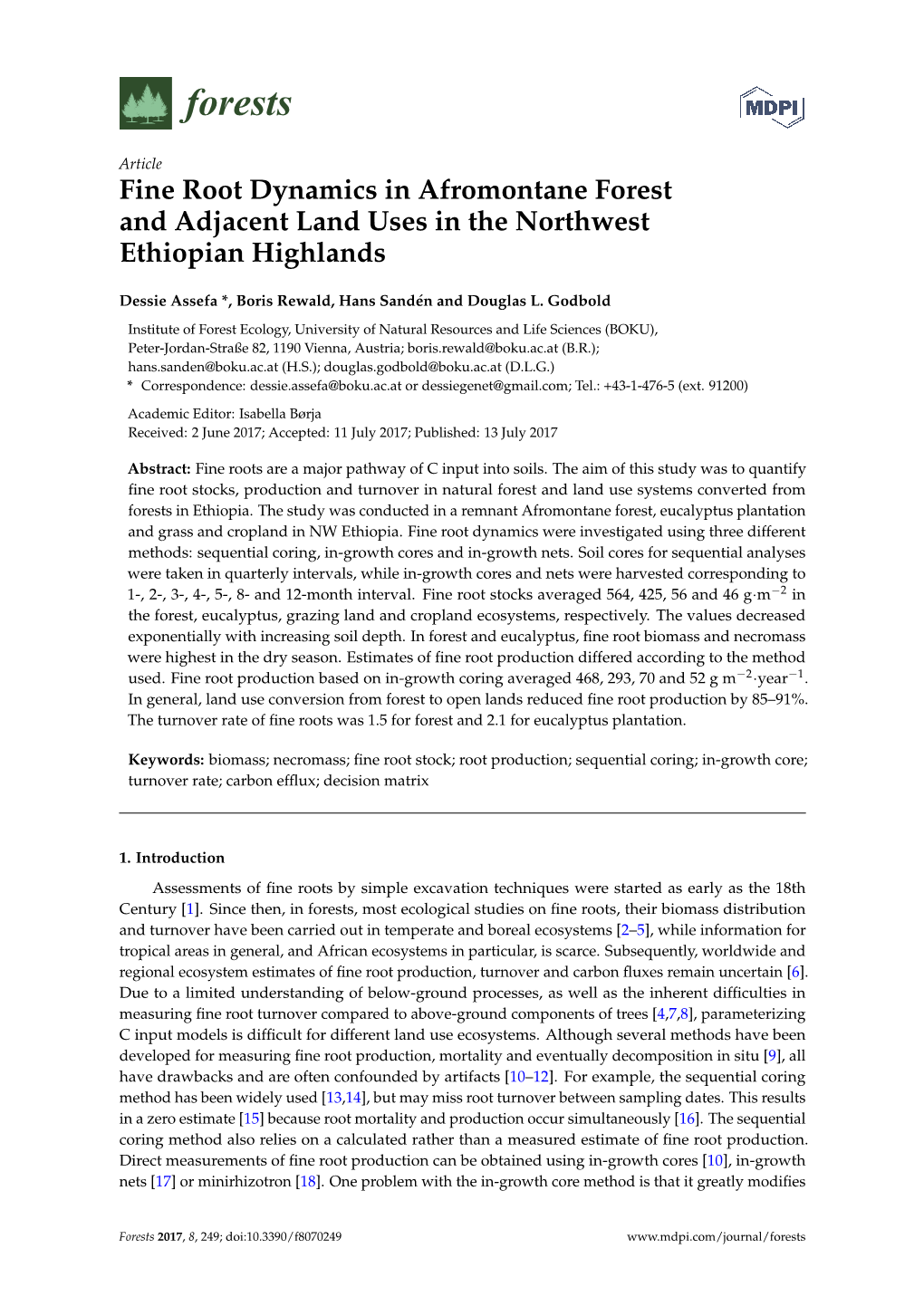
Load more
Recommended publications
-
Mean Annual Temperature Influences Local Fine Root Proliferation and Arbuscular Mycorrhizal Colonization in a Tropical Wet Forest
Received: 4 October 2019 | Revised: 11 May 2020 | Accepted: 21 May 2020 DOI: 10.1002/ece3.6561 ORIGINAL RESEARCH Mean annual temperature influences local fine root proliferation and arbuscular mycorrhizal colonization in a tropical wet forest 1,2 3 4 Suzanne Pierre PhD | Creighton M. Litton PhD | Christian P Giardina PhD | 1 5 Jed P. Sparks PhD | Timothy J. Fahey PhD 1Department of Ecology and Evolutionary Biology, Cornell University, Ithaca, New Abstract York, USA Mean annual temperature (MAT) is an influential climate factor affecting the bio- 2 Department of Integrative Biology, availability of growth-limiting nutrients nitrogen (N) and phosphorus (P). In tropical University of California, Berkeley, Berkeley, California, USA montane wet forests, warmer MAT drives higher N bioavailability, while patterns of 3Department of Natural Resources and P availability are inconsistent across MAT. Two important nutrient acquisition strate- Environmental Management, University of Hawai'i at Manoa, Honolulu, Hawai'i, USA gies, fine root proliferation into bulk soil and root association with arbuscular myc- 4Institute of Pacific Islands Forestry, Pacific orrhizal fungi, are dependent on C availability to the plant via primary production. Southwest Research Station, US Forest The case study presented here tests whether variation in bulk soil N bioavailability Service, Hilo, Hawaii, USA across a tropical montane wet forest elevation gradient (5.2°C MAT range) influences 5Department of Natural Resources, Cornell University, Ithaca, New York, USA (a) morphology fine root proliferation into soil patches with elevated N, P, and N+P relative to background soil and (b) arbuscular mycorrhizal fungal (AMF) colonization Correspondence Suzanne Pierre, Department of Ecology and of fine roots in patches. -
Global Distributions of Arbuscular Mycorrhizal Fungi
Ecosystems (2006) 9: 305–316 DOI: 10.1007/s10021-005-0110-x Global Distributions of Arbuscular Mycorrhizal Fungi Kathleen K. Treseder, * and Alison Crossà Department of Biology, University of Pennsylvania, Philadelphia, Pennsylvania 19104, USA ABSTRACT We examined potential large-scale controls over the in the percentage of plant species that host AM fungi, distribution of arbuscular mycorrhizal (AM) fungi averaging 75%. Contrary to the hypothesis, %RLC, and their host plants. Specifically, we tested the AM abundance, and host plant availability were not hypothesis that AM fungi should be more prevalent related to the size, influx, or turnover rate of soil in biomes where nutrients are primarily present in organic matter pools. Instead, AM abundance was mineral, and not organic, forms. Values of percent- positively correlated with standing stocks of fine age root length colonized (%RLC) by AM fungi, AM roots. The global pool of AM biomass within roots abundance, and host plant availability were com- might approach 1.4 Pg dry weight. We note that piled or calculated from published studies to deter- regions harboring the largest stocks of AM fungi are mine biome-level means. Altogether, 151 also particularly vulnerable to anthropogenic geographic locations and nine biomes were repre- nitrogen deposition, which could potentially alter sented. Percent RLC differed marginally significantly global distributions of AM fungi in the near future. among biomes and was greatest in savannas. AM abundance (defined as total standing root length Key words: arbscular mycorrhizal fungi; below- colonized by AM fungi) varied 63-fold, with lowest ground net primary productivity; fungal biomass; values in boreal forests and highest values in tem- biome; colonization; fine root length; root C:N perate grasslands. -
Fine-Root Responses of Populus Tomentosa Forests to Stand Density
Article Fine-Root Responses of Populus tomentosa Forests to Stand Density Huijuan Bo 1, Chunyan Wen 1, Lianjun Song 2, Yatao Yue 1 and Lishui Nie 1,* 1 College of Forestry, Beijing Forestry University, Beijing 100083, China; [email protected] (H.B.); [email protected] (C.W.); [email protected] (Y.Y.) 2 Huayang Forest Tree Nursery, Xingtai 054700, Hebei, China; [email protected] * Correspondence: [email protected]; Tel.: +86-136-0113-146 Received: 8 July 2018; Accepted: 7 September 2018; Published: 13 September 2018 Abstract: Stand density directly affects the distribution of ecological factors such as light, heat, and water in forest communities and changes the diversity and structure of undergrowth species, thereby affecting soil health. Fine roots can provide water and nutrients to plants rapidly in the fierce competition of soil resources, so as to get rid of environmental factors. This study examined the fine-root responses of the Populus tomentosa clone S86 to three stand densities (plant × row spacing: 2 × 2 m, 4 × 3 m, 4 × 5 m). We measured the biomass, morphology, and nitrogen content of lower- (1–3 order) and higher-order (>3 order) fine roots, and analyzed soil chemical properties in 10–30 cm. The soil from the density (2 × 2 m) stands showed lower soil organic matter content, available nitrogen, available phosphorous, and available potassium than others. Obviously, lower and higher-order fine roots were different: biomass of the >3 order accounted for 77–87% of the total biomass, 1–3-order fine-root diameter around 0.28–0.38 mm, while >3-order fine root were 1.28–1.69 mm; the length of 1–3-order fine root was longer than the >3 order, and root length density, specific root length, and nutrient content between the 1–3 and >3 orders were different. -
Ectomycorrhizal Community Structure and Fi,Mction 2000
Ectomycorrhizal community structure and fi,mction in relation to forest residue harvesting and wood ash applications Shahid Mahmood LUND UNIVERSITY Dissertation 2000 A doctoral thesis at a university in Sweden is produced either as a monograph or as a collection of papers. In the latter case, the introducto~ part constitutes the formal thesis, which summarises the accompanying papers. These have either already been published or are manuscripts at various stages (in press, submitted or in ins). ISBN9!-7105-138-8 sE-LuNBDs/NBME-oo/lo14+l10pp 02000 Shahid Mahmood Cover drawing: Peter Robemtz I DISCLAIMER Portions of this document may be illegible in electronic image products. Images are produced from the best available original document. Organhtion Documentname LUND UNIVERSITY I DOCTORALDISSERTATION Department of Ecology- Mtcrobial Ecology I “eda fday16,20@ Ecology Building, S-223 62 Lund, Sweden I coDfw SE-LUNBDSINBME-OOI1 OI4+I 10 pp Author(a) sponsoringOrgmiration Shahid Mahmood T&feandsubtitls Eotomycorhkal community structure and function in relation to forest residue hawesting and wood ash applications Ectomycorrhizal fungi form symbiotic associations with tree roots and assist in nutrient-uptake and -cycling in forest ecosystems, thereby constitutinga most significantpart of the microbial community. The aims of the studies described in this thesis were to evaluate the potential of DNA-baeed molecular methods in below-ground ectomycorrhizal community studies and to investigate changes in actomycortilzal communities on spruce roots in sites with different N deposition, and in sites subjected to harvesting of forest rasidues or application of wood ash. The ability of selected ectomycorrhizal fungi to mobilise nutriente from wood ash and to colonise root systems in the presence and absence of ash was also studied. -
Fine Root Biomass and Its Relationship with Aboveground Traits of Larix Gmelinii Trees in Northeastern China
Article Fine Root Biomass and Its Relationship with Aboveground Traits of Larix gmelinii Trees in Northeastern China Shengwang Meng 1 ID , Quanquan Jia 2, Guang Zhou 1, Hua Zhou 1, Qijing Liu 1,* and Jian Yu 1 1 College of Forestry, Beijing Forestry University, Beijing 100083, China; [email protected] (S.M.); [email protected] (G.Z.); [email protected] (H.Z.); [email protected] (J.Y.) 2 Jiangxi Academy of Forestry, Nanchang 330032, China; [email protected] * Correspondence: [email protected]; Tel.: +010-6233-8133 Received: 23 November 2017; Accepted: 12 January 2018; Published: 16 January 2018 Abstract: Fine roots play a prominent role in forest carbon flux, nutrient and water acquisition; however, information on fine roots remains scarce due to the limitation of measuring methods. In this study, a nested regression method was used to estimate the biomass and surface area of fine roots of individual Larix gmelinii trees that dominate northernmost China. Aboveground traits including leaf biomass, leaf area, stem volume and aboveground biomass were also investigated. In particular, the relationships between leaves and fine roots, in terms of biomass and area, were examined. The results revealed that allometric models of fine roots, total roots, and leaves consistently fit well with Adj. R2 = 0.92–0.97. The root-shoot ratio at the individual tree level was approximately 0.28. There were robust positive linear correlations between absorption (fine root biomass, fine root surface area) and production (leaf biomass, leaf area) (Adj. R2 = 0.95, p < 0.001). In conclusion, the close coupling between fine roots and leaves presented in this study provides support for the theory of functional equilibrium. -
Tamm Review Deep Fine Roots in Forest Ecosystems Why Dig Deeper?
Forest Ecology and Management 466 (2020) 118135 Contents lists available at ScienceDirect Forest Ecology and Management journal homepage: www.elsevier.com/locate/foreco Tamm Review: Deep fine roots in forest ecosystems: Why dig deeper? T ⁎ Amandine Germona,b, Jean-Paul Laclaua,b,c, Agnès Robinb,c,d, Christophe Jourdanb,c, a UNESP, São Paulo State University, School of Agricultural Sciences, Botucatu, São Paulo 18610-307, Brazil b Eco&Sols, Univ Montpellier, CIRAD, INRA, IRD, Montpellier SupAgro, Montpellier, France c CIRAD, UMR Eco&Sols, F-34060 Montpellier, France d ESALQ, Soil Science Department, Escola Superior de Agricultura, Luiz de Queiroz, Universidade de São Paulo, CEP 13418-900 Piracicaba, SP, Brazil ARTICLE INFO ABSTRACT Keywords: While the number of studies dealing with fine root dynamics in deep soils layers (depth > 1 m) has increased Deep rooting sharply recently, the phenology, the morphology, the anatomy and the role of deep fine roots are still poorly Root growth known in forest ecosystems. This review summarizes the current knowledge on fine root production, mortality Root traits and longevity in deep soil layers, mycorrhizal association with deep roots, and the role of deep fine roots on Carbon sequestration carbon, water and nutrient cycling in forest ecosystems. Plant species are known to be more deeply rooted in Water uptake tropical ecosystems than in temperate and boreal ecosystems, but deep-rooted species are common in a wide Nutrient uptake Deep mycorrhizas range of climates. Deep fine roots are highly plastic in response to changes in environmental conditions andsoil resources. Recent studies show that functional traits can be different for deep and shallow roots, with a possible functional specialization of deep fine roots to take up nutrients. -
New Approach for Capturing Soluble Root Exudates in Forest Soils
Functional Ecology 2008, 22, 990–999 doi: 10.1111/j.1365-2435.2008.01495.x BELOWGROUNDBlackwell Publishing Ltd RESPONSES TO CLIMATE CHANGE New approach for capturing soluble root exudates in forest soils Richard P. Phillips*, Yael Erlitz, Raven Bier and Emily S. Bernhardt Department of Biology, Duke University, Durham, NC 27708, USA Summary 1. Soluble root exudates are notoriously difficult to collect in non-hydroponic systems because they are released in a narrow zone around roots and are rapidly assimilated by rhizosphere microbes. This has substantially limited our understanding of their rates of release and chemical composition in situ, and by extension, their ecological significance. 2. Here we describe the advantages and limitations of several commonly employed methods for measuring exudation with respect to their potential adaptability for field use in forest ecosystems. Then, we introduce a novel in situ method for measuring exudation in forest soils, and present preliminary results of the spatial and temporal dynamics of loblolly pine (Pinus taeda L.) exudation at the Duke Forest FACTS-1 site, North Carolina, USA from April 2007 to July 2008. 3. Exudation rates varied by an order of magnitude, with the highest rates occurring in late-June 2007 and mid-July 2008, and the lowest rates occurring during late-August 2007. On an annual basis, we estimate pine roots in the upper 15 cm of soil release c. 9 g C m–2 year–1 via this flux, which represents 1–2% of net primary productivity at the site. 4. The magnitude of exudation rates did not differ across an N availability gradient but did track general patterns of below-ground C allocation at the site. -
Root Exudation Rates Decrease with Increasing Latitude in Some Tree Species
Article Root Exudation Rates Decrease with Increasing Latitude in Some Tree Species Liu Yang 1 , Xiuwei Wang 1,* , Zijun Mao 2, Zhiyan Jiang 1, Yang Gao 2, Xiangwei Chen 1 and Doug P. Aubrey 3,4 1 Key Laboratory of Sustainable Forest Ecosystem Management-Ministry of Education, Northeast Forestry University, Harbin 150040, China; [email protected] (L.Y.); [email protected] (Z.J.); [email protected] (X.C.) 2 College of Chemistry, Chemical Engineering and Resource Utilization, Northeast Forestry University, Harbin 150040, China; [email protected] (Z.M.); [email protected] (Y.G.) 3 Savannah River Ecology Laboratory, University of Georgia, PO Drawer E, Aiken, SC 29802, USA; [email protected] 4 Warnell School of Forestry and Natural Resources, University of Georgia, 180 E. Green St., Athens, GA 30602, USA * Correspondence: [email protected]; Tel.: +86-451-8219-1829 Received: 17 August 2020; Accepted: 27 September 2020; Published: 28 September 2020 Abstract: Research Highlights: Understanding of the spatial variation of root exudation on a regional scale can help understand the response of plant physiological activities to environmental changes. Background and Objectives: Although root exudation has become an important topic in belowground ecology, its relationship with root traits and environmental factors is poorly understood. Our objective was to explore how root traits and environmental factors influence root exudation. Materials and Methods: We used a multi-factorial design consisting of three tree species spanning across sites located at three latitudes to assess root exudation dynamics, which was measured using a syringe-basis incubation system. Results: The strongest and clearest effect observed in our study was a decrease in root exudation rates of Korean pine (Pinus koraiensis Sieb. -
Fine-Root Ecology Database (FRED): a Global Collection of Root Trait
Fine-Root Ecology Database (FRED): A Global Collection of Root Trait Data with Coincident Site, Vegetation, Edaphic, and Climactic Data, Version 2 Document Revision Date: 31 May 2018 Summary: To address the need for a centralized root trait database, we compiled the Fine-Root Ecology Database (FRED) from published and unpublished data sources. We have continued to add to the FRED database since the release of FRED 1.0, and a new version of FRED is now available. FRED 2.0 houses 50% more data, with more than 105,000 observations of root traits; ancillary data on associated site, vegetation, edaphic, and climatic conditions from across the globe have also increased concurrently. FRED is focused on fine roots (roots less than 2 mm in diameter), as coarse roots are studied using different methodology, often at very different scales, and have different traits and trait interpretations. However, FRED accepts data collected from roots of all sizes, and already contains several observations of coarse roots. Data collection will continue for the foreseeable future. Related Publications: More details about FRED and the motivation for undertaking this monumental data compilation can be found in: Iversen CM, McCormack ML, Powell AS, Blackwood CB, Freschet GT, Kattge J, Roumet C, Stover DB, Soudzilovskaia NA, Valverde-Barrantes OJ, van Bodegom PM, Violle C. 2017. Viewpoints: A global Fine-Root Ecology Database to address belowground challenges in plant ecology. New Phytologist 215: 15-26. https://doi.org/10.1111/nph.14486 McCormack ML, Powell AS, Iversen CM. 2018. Letter: The Fine-Root Ecology Database version 2 - bigger, better, and free. -
Ectomycorrhizal Colonisation in Declining Oak Stands on the Krotoszyn Plateau, Poland
Article Ectomycorrhizal Colonisation in Declining Oak Stands on the Krotoszyn Plateau, Poland Roman Mariusz Bzdyk 1, Jacek Olchowik 2,*, Marcin Studnicki 3 , Justyna Anna Nowakowska 4 , Tomasz Oszako 5 , Alexander Urban 6 and Dorota Hilszcza ´nska 1 1 Department of Forest Ecology, Forest Research Institute, Braci Le´snej3, 05-090 S˛ekocinStary, Poland; [email protected] (R.M.B.); [email protected] (D.H.) 2 Department of Plant Pathology, Faculty of Horticulture, Biotechnology and Landscape Architecture, Warsaw University of Life Sciences, Nowoursynowska 159, 02-776 Warsaw, Poland 3 Department of Experimental Design and Bioinformatics, Faculty of Agriculture and Biology, Warsaw University of Life Sciences, Nowoursynowska 159, 02-776 Warsaw, Poland; [email protected] 4 Faculty of Biology and Environmental Sciences, Cardinal Stefan Wyszynski University in Warsaw, Wóycickiego 1/3, 01-938 Warsaw, Poland; [email protected] 5 Department of Forest Protection, Forest Research Institute, Braci Le´snej3, 05-090 S˛ekocinStary, Poland; [email protected] 6 Department of Botany and Biodiversity Research, Faculty of Life Sciences, University of Vienna, Rennweg 14, Wien A-1030, Austria; [email protected] * Correspondence: [email protected]; Tel.: +48-790-581-350 Received: 5 December 2018; Accepted: 31 December 2018; Published: 4 January 2019 Abstract: We describe the ectomycorrhizal (ECM) root tips and the diversity of mycorrhizal fungal species at three English oak (Quercus robur) sites (two 120 year old sites and one 60 year old site). The three oak stands in decline, located in western Poland, were characterized by a low degree of vital ECM colonization: 30.2%, 29.1% and 25.6% at Krotoszyn (K), Piaski (P) and Karczma Borowa (KB), respectively. -
Global Patterns in Fine Root Decomposition: Climate, Chemistry
Ecology Letters, (2019) 22: 946–953 doi: 10.1111/ele.13248 LETTER Global patterns in fine root decomposition: climate, chemistry, mycorrhizal association and woodiness Abstract Craig R. See,1* Fine root decomposition constitutes a critical yet poorly understood flux of carbon and nutrients Michael Luke McCormack,2,3 in terrestrial ecosystems. Here, we present the first large-scale synthesis of species trait effects on Sarah E. Hobbie,1 the early stages of fine root decomposition at both global and local scales. Based on decomposi- Habacuc Flores-Moreno,1,4 tion rates for 279 plant species across 105 studies and 176 sites, we found that mycorrhizal associ- Whendee L. Silver5 and ation and woodiness are the best categorical traits for predicting rates of fine root decomposition. Peter G. Kennedy1,2 Consistent positive effects of nitrogen and phosphorus concentrations and negative effects of lig- nin concentration emerged on decomposition rates within sites. Similar relationships were present across sites, along with positive effects of temperature and moisture. Calcium was not consistently related to decomposition rate at either scale. While the chemical drivers of fine root decomposi- tion parallel those of leaf decomposition, our results indicate that the best plant functional groups for predicting fine root decomposition differ from those predicting leaf decomposition. Keywords Carbon cycling, decay, MANE framework, meta-analysis, nutrient cycling, organic matter, plant economics spectrum, plant functional traits, plant litter, soil organic matter. Ecology Letters (2019) 22: 946–953 and chemical composition (Iversen et al. 2017) and are INTRODUCTION ephemeral in comparison to structural tissues (Eissenstat & Plant litter decomposition in terrestrial systems constitutes Yanai 1997; McCormack et al. -
The Response of Fine Root Morphological and Physiological Traits to Added Nitrogen in Schrenk's Spruce
The response of fine root morphological and physiological traits to added nitrogen in Schrenk’s spruce (Picea schrenkiana)of the Tianshan mountains, China Lu Gong1,2,* and Jingjing Zhao1,2,* 1 Key Laboratory of Oasis Ecology, Xinjiang University, Urumqi, China 2 College Resources and Environment Science, Xinjiang University, Urumqi, China * These authors contributed equally to this work. ABSTRACT Fine roots are essential for water and nutrient uptake in plants, but little is known about the variation in fine root traits and the underlying mechanisms that drive it. Understanding the responses of fine root function traits to changing environmental conditions and the role of fine root traits as drivers of forest ecosystem processes are critical for informing physiological and ecological theory as well as ecosystem management. We measured morphological and physiological traits of fine roots from six soil layers and three diameter classes in Schrenk’s spruce (Picea shrenkiana) forests of the Tianshan mountains, China. We found significant effects of nitrogen addition on these morphological and physiological traits, which varied by soil layer and root diameter. Specifically, specific root length (SRL) was higher in medium N addition group (N2) than in control group (N0). Specific root area (SRA) was higher in the control group (N0) than fertilized groups (N1, N2 and N3). Root tissue density (RTD) was higher in low N addition group (N1) than in the other group. Root dry matter content had no significant difference among four treatment groups. SRL, SRA, and RTD of fine roots in different diameter classes were all significantly different between high N addition (N3) and the control (N0) groups.