Taphonomy of a Lance Formation(Maastrichtian, WY) Dinosaur Bonebed with a Focus on Tooth Traces Matthew A
Total Page:16
File Type:pdf, Size:1020Kb
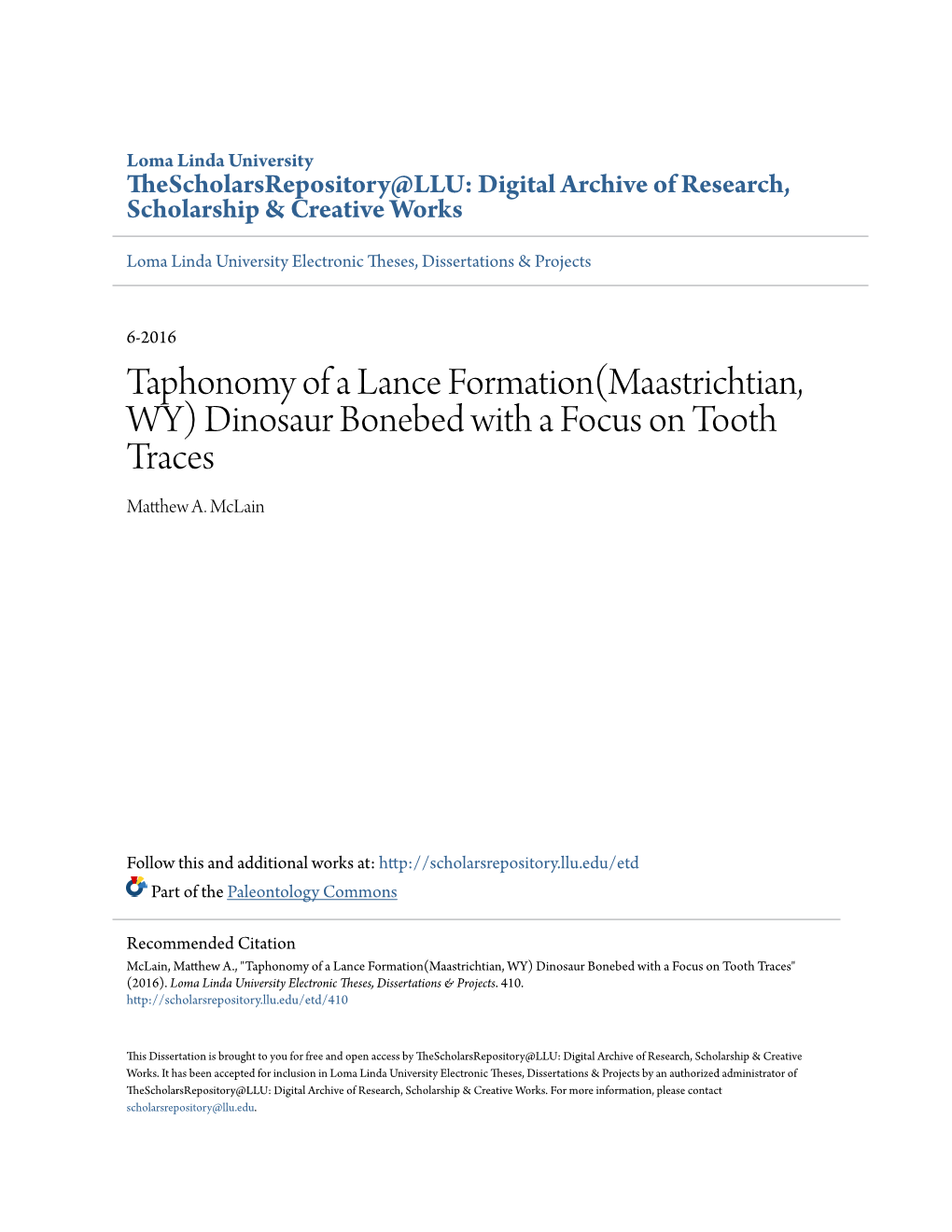
Load more
Recommended publications
-
Washakie Basin Wamsutter Arch SUBSURFACE STRATIGRAPHIC CROSS SECTION of CRETACEOUS and LOWER TERTIARY ROCKS in the SOUTHWESTERN
U.S. DEPARTMENT OF THE INTERIOR DIGITAL DATA SERIES DDS–69–D U.S. GEOLOGICAL SURVEY CHAPTER 14, PLATE 1–2 Washakie Basin Wamsutter arch 23 East 24 e r e Amoco Production Company h t u Frewen Deep Unit 1 c 22 26 C SE sec. 13, T. 19 N., R. 95 W. KB 6,937 Amoco Production Company 25 Tierney Unit 2 4.0 mi NW NE sec. 15, T. 19 N., R. 94 W. Amoco Production Company 7.0 mi KB 6,691 composite log 1 Tipton Unit II Amoco Production Company SE NW sec. 14, T. 19 N., R. 96 W. 8.5 mi Amoco Production Company Amoco-Champlin 278 E-3 500 27 e KB 6,973 GR Res. Echo Springs Deep 1 NE SW sec. 13, T. 18 N., R. 93 W. ) t n r NW SW sec. 21, T. 18 N., R. 93 W. KB 6,874 e a KB 6,783 Amoco Production Company c p o 3.0 mi Amoco-Champlin 278 E-1 ( E 1000 SE SE sec. 13, T. 18 N., R. 93 W. Amoco Production Company GR KB 6,936 3.2 mi Creston Nose 1 Wasatch and Green River NE SW sec. 9, T. 18 N., R. 92 W. 6.0 mi Formations undivided 1000 500 Res. GR Res. KB 7,001 ? 21 1500 ? ? ? 1500 1000 6.7 mi ? 500 P re s GR Res. e Union Pacific Resources n 2000 t -d Sidewinder 1 H Overland a ? y NW NW sec. 2, T. 19 N., R. -
Redalyc.Preliminary Report on a Late Cretaceous Vertebrate Fossil
Boletín de la Sociedad Geológica Mexicana ISSN: 1405-3322 [email protected] Sociedad Geológica Mexicana, A.C. México Rivera-Sylva, Héctor E.; Frey, Eberhard; Palomino-Sánchez, Francisco J.; Guzmán-Gutiérrez, José Rubén; Ortiz-Mendieta, Jorge A. Preliminary Report on a Late Cretaceous Vertebrate Fossil Assemblage in Northwestern Coahuila, Mexico Boletín de la Sociedad Geológica Mexicana, vol. 61, núm. 2, 2009, pp. 239-244 Sociedad Geológica Mexicana, A.C. Distrito Federal, México Available in: http://www.redalyc.org/articulo.oa?id=94316034014 How to cite Complete issue Scientific Information System More information about this article Network of Scientific Journals from Latin America, the Caribbean, Spain and Portugal Journal's homepage in redalyc.org Non-profit academic project, developed under the open access initiative Preliminary Report on a Late Cretaceous Vertebrate Fossil Assemblage in Northwestern Coahuila, Mexico 239 BOLETÍN DE LA SOCIEDAD GEOLÓGICA MEXICANA VOLUMEN 61, NÚM. 2, 2009, P. 239-244 D GEOL DA Ó E G I I C C O A S 1904 M 2004 . C EX . ICANA A C i e n A ñ o s Preliminary Report on a Late Cretaceous Vertebrate Fossil Assemblage in Northwestern Coahuila, Mexico Héctor E. Rivera-Sylva1, Eberhard Frey2, Francisco J. Palomino-Sánchez3, José Rubén Guzmán-Gutiérrez4, Jorge A. Ortiz-Mendieta5 1 Departamento de Paleontología, Museo del Desierto. Pról. Pérez Treviño 3745, 25015, Saltillo, Coah., México. 2 Geowissenschaftliche Abteilung,Staatliches Museum für Naturkunde Karlsruhe. Karlsruhe, Alemania. 3 Laboratorio de Petrografía y Paleontología, Instituto Nacional de Estadística, Geografía e Informática, Aguascalientes, Ags., México. 4 Centro para la Conservación del Patrimonio Natural y Cultural de México, Aguascalientes, Ags., México. -
Structural Interpretation of the Cherokee Arch, South
STRUCTURAL INTERPRETATION OF THE CHEROKEE ARCH, SOUTH CENTRAL WYOMING, USING 3-D SEISMIC DATA AND WELL LOGS by Joel Ysaccis B. ABSTRACT The purpose of this study is to use 3-D seismic data and well logs to map the structural evolution of the Cherokee Arch, a major east-west trending basement high along the Colorado-Wyoming state line. The Cherokee Arch lies along the Cheyenne lineament, a major discontinuity or suture zone in the basement. Recurrent, oblique-slip offset is interpreted to have occurred along faults that make up the arch. Gas fields along the Cherokee Arch produce from structural and structural-stratigraphic traps, mainly in Cretaceous rocks. Some of these fields, like the South Baggs – West Side Canal fields, have gas production from multiple pays. The tectonic evolution of the Cherokee Arch has not been previously studied in detail. About 315 mi2 (815 km2) of 3-D seismic data were analyzed in this study to better understand the kinematic evolution of the area. The interpretation involved mapping the Madison, Shinarump, Above Frontier, Mancos, Almond, Lance/Fox Hills and Fort Union horizons, as well as defining fault geometries. Structure maps on these horizons show the general tendency of the structure to dip towards the west. The Cherokee Arch is an asymmetrical anticline in the hanging wall, which is mainly transected by a south-dipping series of east-west striking thrust faults. The interpreted thrust faults generally terminate within the Mancos to Above Frontier interval, and their vertical offset increases in magnitude down to the basement. Post-Mancos iii intervals are dominated by near-vertical faults with apparent normal offset. -
What Can Be Learned from a Fossil Tooth? an Inquiry Into the Life & Diet of a Small Theropod Dinosaur
What Can Be Learned From A Fossil Tooth? An Inquiry Into The Life & Diet Of A Small Theropod Dinosaur Karolyn Hamlin The Hell Creek and Lance Formations of the animals alive at the time, paleontologists Montana, North Dakota, South Dakota, and strive to understand why the K-Pg extinction Wyoming (Fig. 1) are valuable sources of seemed to affect dinosaurs so heavily Maastrichtian age (Late Cretaceous) fossils. compared to other organisms. Diverse The Maastrichtian is the last age before the vertebrate remains have been found at these well-known Cretaceous-Paleogene (K-Pg) sites, including fish, sharks, amphibians, extinction that marked the demise of the non- mammals, reptiles, and birds. The phrase avian dinosaurs. Studying the fossils of these “non-avian dinosaurs” hints that birds are formations is not only key to understanding considered dinosaurs, the avian dinosaurs, and the effects the K-Pg extinction had on this paper will focus on the group that biodiversity, but understand the biodiversity includes birds, their close ancestors, and near trends leading up to it. Through understanding cousins: the theropods. these trends as well as the characteristics of . Figure 1: Map of the Hell Creek and Lance Formations. (Modified from Johnson, 1992) 53 Figure 2: Recreation of the Late Cretaceous Western Interior Seaway. The Hell Creek and Lance Formations cover areas that were very near water, making fish available as prey animals. (Blakey, 2006) If you have ever been to a museum with a mouths that flat teeth for grinding are useful dinosaur exhibit, you may have seen a for consuming fibrous plant material, and recreation of a large theropod such as sharp teeth for puncturing or shearing are Tyrannosaurus rex or Allosaurus. -
Forgotten Crocodile from the Kirtland Formation, San Juan Basin, New
posed that the narial cavities of Para- Wima1l- saurolophuswere vocal resonating chambers' Goniopholiskirtlandicus Apparently included with this material shippedto Wiman was a partial skull that lromthe Wiman describedas a new speciesof croc- forgottencrocodile odile, Goniopholis kirtlandicus. Wiman publisheda descriptionof G. kirtlandicusin Basin, 1932in the Bulletin of the GeologicalInstitute KirtlandFormation, San Juan of IJppsala. Notice of this specieshas not appearedin any Americanpublication. Klilin NewMexico (1955)presented a descriptionand illustration of the speciesin French, but essentially repeatedWiman (1932). byDonald L. Wolberg, Vertebrate Paleontologist, NewMexico Bureau of lVlinesand Mineral Resources, Socorro, NIM Localityinformation for Crocodilian bone, armor, and teeth are Goni o p holi s kir t landicus common in Late Cretaceous and Early Ter- The skeletalmaterial referred to Gonio- tiary deposits of the San Juan Basin and pholis kirtlandicus includesmost of the right elsewhere.In the Fruitland and Kirtland For- side of a skull, a squamosalfragment, and a mations of the San Juan Basin, Late Creta- portion of dorsal plate. The referral of the ceous crocodiles were important carnivores of dorsalplate probably represents an interpreta- the reconstructed stream and stream-bank tion of the proximity of the material when community (Wolberg, 1980). In the Kirtland found. Figs. I and 2, taken from Wiman Formation, a mesosuchian crocodile, Gonio- (1932),illustrate this material. pholis kirtlandicus, discovered by Charles H. Wiman(1932, p. 181)recorded the follow- Sternbergin the early 1920'sand not described ing locality data, provided by Sternberg: until 1932 by Carl Wiman, has been all but of Crocodile.Kirtland shalesa 100feet ignored since its description and referral. "Skull below the Ojo Alamo Sandstonein the blue Specimensreferred to other crocodilian genera cley. -
Theropod Teeth from the Upper Maastrichtian Hell Creek Formation “Sue” Quarry: New Morphotypes and Faunal Comparisons
Theropod teeth from the upper Maastrichtian Hell Creek Formation “Sue” Quarry: New morphotypes and faunal comparisons TERRY A. GATES, LINDSAY E. ZANNO, and PETER J. MAKOVICKY Gates, T.A., Zanno, L.E., and Makovicky, P.J. 2015. Theropod teeth from the upper Maastrichtian Hell Creek Formation “Sue” Quarry: New morphotypes and faunal comparisons. Acta Palaeontologica Polonica 60 (1): 131–139. Isolated teeth from vertebrate microfossil localities often provide unique information on the biodiversity of ancient ecosystems that might otherwise remain unrecognized. Microfossil sampling is a particularly valuable tool for doc- umenting taxa that are poorly represented in macrofossil surveys due to small body size, fragile skeletal structure, or relatively low ecosystem abundance. Because biodiversity patterns in the late Maastrichtian of North American are the primary data for a broad array of studies regarding non-avian dinosaur extinction in the terminal Cretaceous, intensive sampling on multiple scales is critical to understanding the nature of this event. We address theropod biodiversity in the Maastrichtian by examining teeth collected from the Hell Creek Formation locality that yielded FMNH PR 2081 (the Tyrannosaurus rex specimen “Sue”). Eight morphotypes (three previously undocumented) are identified in the sample, representing Tyrannosauridae, Dromaeosauridae, Troodontidae, and Avialae. Noticeably absent are teeth attributed to the morphotypes Richardoestesia and Paronychodon. Morphometric comparison to dromaeosaurid teeth from multiple Hell Creek and Lance formations microsites reveals two unique dromaeosaurid morphotypes bearing finer distal denticles than present on teeth of similar size, and also differences in crown shape in at least one of these. These findings suggest more dromaeosaurid taxa, and a higher Maastrichtian biodiversity, than previously appreciated. -
Stratigraphy of the Upper Cretaceous Fox Hills Sandstone and Adjacent
Stratigraphy of the Upper Cretaceous Fox Hills Sandstone and AdJa(-erit Parts of the Lewis Sliale and Lance Formation, East Flank of the Rock Springs Uplift, Southwest lo U.S. OEOLOGI AL SURVEY PROFESSIONAL PAPER 1532 Stratigraphy of the Upper Cretaceous Fox Hills Sandstone and Adjacent Parts of the Lewis Shale and Lance Formation, East Flank of the Rock Springs Uplift, Southwest Wyoming By HENRYW. ROEHLER U.S. GEOLOGICAL SURVEY PROFESSIONAL PAPER 1532 Description of three of/lapping barrier shorelines along the western margins of the interior seaway of North America UNITED STATES GOVERNMENT PRINTING OFFICE, WASHINGTON : 1993 U.S. DEPARTMENT OF THE INTERIOR BRUCE BABBITT, Secretary U.S. GEOLOGICAL SURVEY Dallas L. Peck, Director Any use of trade, product, or firm names in this publication is for descriptive purposes only and does not imply endorsement by the U.S. Government. Library of Congress Cataloging-in-Publication Data Roehler, Henry W. Stratigraphy of the Upper Cretaceous Fox Hills sandstone and adjacent parts of the Lewis shale and Lance formation, east flank of the Rock Springs Uplift, southwest Wyoming / by Henry W. Roehler. p. cm. (U.S. Geological Survey professional paper ; 1532) Includes bibliographical references. Supt.ofDocs.no.: I19.16:P1532 1. Geology, Stratigraphic Cretaceous. 2. Geology Wyoming. 3. Fox Hills Formation. I. Geological Survey (U.S.). II. Title. III. Series. QE688.R64 1993 551.7T09787 dc20 92-36645 CIP For sale by USGS Map Distribution Box 25286, Building 810 Denver Federal Center Denver, CO 80225 CONTENTS Page Page Abstract......................................................................................... 1 Stratigraphy Continued Introduction................................................................................... 1 Formations exposed on the east flank of the Rock Springs Description and accessibility of the study area ................ -
The Geology of New Mexico As Understood in 1912: an Essay for the Centennial of New Mexico Statehood Part 2 Barry S
Celebrating New Mexico's Centennial The geology of New Mexico as understood in 1912: an essay for the centennial of New Mexico statehood Part 2 Barry S. Kues, Department of Earth and Planetary Sciences, University of New Mexico, Albuquerque, New Mexico, [email protected] Introduction he first part of this contribution, presented in the February Here I first discuss contemporary ideas on two fundamental areas 2012 issue of New Mexico Geology, laid the groundwork for an of geologic thought—the accurate dating of rocks and the move- exploration of what geologists knew or surmised about the ment of continents through time—that were at the beginning of Tgeology of New Mexico as the territory transitioned into statehood paradigm shifts around 1912. Then I explore research trends and in 1912. Part 1 included an overview of the demographic, economic, the developing state of knowledge in stratigraphy and paleontol- social, cultural, and technological attributes of New Mexico and its ogy, two disciplines of geology that were essential in understand- people a century ago, and a discussion of important individuals, ing New Mexico’s rock record (some 84% of New Mexico’s surface institutions, and areas and methods of research—the geologic envi- area is covered by sediments or sedimentary rocks) and which were ronment, so to speak—that existed in the new state at that time. advancing rapidly through the first decade of the 20th century. The geologic time scale and age of rocks The geologic time scale familiar to geologists working in New The USGS did not adopt the Paleocene as the earliest epoch of the Mexico in 1912 was not greatly different from that used by modern Cenozoic until 1939. -
71St Annual Meeting Society of Vertebrate Paleontology Paris Las Vegas Las Vegas, Nevada, USA November 2 – 5, 2011 SESSION CONCURRENT SESSION CONCURRENT
ISSN 1937-2809 online Journal of Supplement to the November 2011 Vertebrate Paleontology Vertebrate Society of Vertebrate Paleontology Society of Vertebrate 71st Annual Meeting Paleontology Society of Vertebrate Las Vegas Paris Nevada, USA Las Vegas, November 2 – 5, 2011 Program and Abstracts Society of Vertebrate Paleontology 71st Annual Meeting Program and Abstracts COMMITTEE MEETING ROOM POSTER SESSION/ CONCURRENT CONCURRENT SESSION EXHIBITS SESSION COMMITTEE MEETING ROOMS AUCTION EVENT REGISTRATION, CONCURRENT MERCHANDISE SESSION LOUNGE, EDUCATION & OUTREACH SPEAKER READY COMMITTEE MEETING POSTER SESSION ROOM ROOM SOCIETY OF VERTEBRATE PALEONTOLOGY ABSTRACTS OF PAPERS SEVENTY-FIRST ANNUAL MEETING PARIS LAS VEGAS HOTEL LAS VEGAS, NV, USA NOVEMBER 2–5, 2011 HOST COMMITTEE Stephen Rowland, Co-Chair; Aubrey Bonde, Co-Chair; Joshua Bonde; David Elliott; Lee Hall; Jerry Harris; Andrew Milner; Eric Roberts EXECUTIVE COMMITTEE Philip Currie, President; Blaire Van Valkenburgh, Past President; Catherine Forster, Vice President; Christopher Bell, Secretary; Ted Vlamis, Treasurer; Julia Clarke, Member at Large; Kristina Curry Rogers, Member at Large; Lars Werdelin, Member at Large SYMPOSIUM CONVENORS Roger B.J. Benson, Richard J. Butler, Nadia B. Fröbisch, Hans C.E. Larsson, Mark A. Loewen, Philip D. Mannion, Jim I. Mead, Eric M. Roberts, Scott D. Sampson, Eric D. Scott, Kathleen Springer PROGRAM COMMITTEE Jonathan Bloch, Co-Chair; Anjali Goswami, Co-Chair; Jason Anderson; Paul Barrett; Brian Beatty; Kerin Claeson; Kristina Curry Rogers; Ted Daeschler; David Evans; David Fox; Nadia B. Fröbisch; Christian Kammerer; Johannes Müller; Emily Rayfield; William Sanders; Bruce Shockey; Mary Silcox; Michelle Stocker; Rebecca Terry November 2011—PROGRAM AND ABSTRACTS 1 Members and Friends of the Society of Vertebrate Paleontology, The Host Committee cordially welcomes you to the 71st Annual Meeting of the Society of Vertebrate Paleontology in Las Vegas. -
The Dinosaur Park - Bearpaw Formation Transition in the Cypress Hills Region of Southwestern Saskatchewan, Canada Meagan M
The Dinosaur Park - Bearpaw Formation Transition in the Cypress Hills Region of Southwestern Saskatchewan, Canada Meagan M. Gilbert Department of Geological Sciences, University of Saskatchewan; [email protected] Summary The Upper Cretaceous Dinosaur Park Formation (DPF) is a south- and eastward-thinning fluvial to marginal marine clastic-wedge in the Western Canadian Sedimentary Basin. The DPF is overlain by the Bearpaw Formation (BF), a fully marine clastic succession representing the final major transgression of the epicontinental Western Interior Seaway (WIS) across western North America. In southwestern Saskatchewan, the DPF is comprised of marginal marine coal, carbonaceous shale, and heterolithic siltstone and sandstone grading vertically into marine sandstone and shale of the Bearpaw Formation. Due to Saskatchewan’s proximity to the paleocoastline, 5th order transgressive cycles resulted in the deposition of multiple coal seams (Lethbridge Coal Zone; LCZ) in the upper two-thirds of the DPF in the study area. The estimated total volume of coal is 48109 m3, with a gas potential of 46109 m3 (Frank, 2005). The focus of this study is to characterize the facies and facies associations of the DPF, the newly erected Manâtakâw Member, and the lower BF in the Cypress Hills region of southwestern Saskatchewan utilizing core, outcrop, and geophysical well log data. This study provides a comprehensive sequence stratigraphic overview of the DPF-BF transition in Saskatchewan and the potential for coalbed methane exploration. Introduction The Dinosaur Park and Bearpaw Formations in Alberta, and its equivalents in Montana, have been the focus of several sedimentologic and stratigraphic studies due to exceptional outcrop exposure and extensive subsurface data (e.g., McLean, 1971; Wood, 1985, 1989; Eberth and Hamblin, 1993; Tsujita, 1995; Catuneanu et al., 1997; Hamblin, 1997; Rogers et al., 2016). -
A Juvenile Cf. Edmontosaurus Annectens (Ornithischia, Hadrosauridae) Femur Documents a Previously Unreported Intermediate Growth Stage for This Taxon Andrew A
Vertebrate Anatomy Morphology Palaeontology 7:59–67 59 ISSN 2292-1389 A juvenile cf. Edmontosaurus annectens (Ornithischia, Hadrosauridae) femur documents a previously unreported intermediate growth stage for this taxon Andrew A. Farke1,2,3,* and Eunice Yip2 1Raymond M. Alf Museum of Paleontology, 1175 West Baseline Road, Claremont, CA, 91711, USA 2The Webb Schools, 1175 West Baseline Road, Claremont, CA, 91711, USA 3Dinosaur Institute, Natural History Museum of Los Angeles County, 900 Exposition Blvd. Los Angeles, CA, 90007, USA; [email protected] Abstract: A nearly complete, but isolated, femur of a small hadrosaurid from the Hell Creek Formation of Montana is tentatively referred to Edmontosaurus annectens. At 28 cm long, the element can be classified as likely that from an ‘early juvenile’ individual, approximately 24% of the maximum known femur length for this species. Specimens from this size range and age class have not been described previously for E. annectens. Notable trends with increasing body size include increasingly distinct separation of the femoral head and greater trochanter, relative increase in the size of the cranial trochanter, a slight reduction in the relative breadth of the fourth trochanter, and a relative increase in the prominence of the cranial intercondylar groove. The gross profile of the femoral shaft is fairly consistent between the smallest and largest individuals. Although an ontogenetic change from relatively symmetrical to an asymmetrical shape in the fourth trochanter has been suggested previously, the new juvenile specimen shows an asymmetric fourth tro- chanter. Thus, there may not be a consistent ontogenetic pattern in trochanteric morphology. An isometric relationship between femoral circumference and femoral length is confirmed for Edmontosaurus. -
Histology and Ontogeny of Pachyrhinosaurus Nasal Bosses By
Histology and Ontogeny of Pachyrhinosaurus Nasal Bosses by Elizabeth Kruk A thesis submitted in partial fulfillment of the requirements for the degree of Master of Science in Systematics and Evolution Department of Biological Sciences University of Alberta © Elizabeth Kruk, 2015 Abstract Pachyrhinosaurus is a peculiar ceratopsian known only from Upper Cretaceous strata of Alberta and the North Slope of Alaska. The genus consists of three described species Pachyrhinosaurus canadensis, Pachyrhinosaurus lakustai, and Pachyrhinosaurus perotorum that are distinguishable by cranial characteristics, including parietal horn shape and orientation, absence/presence of a rostral comb, median parietal bar horns, and profile of the nasal boss. A fourth species of Pachyrhinosaurus is described herein and placed into its phylogenetic context within Centrosaurinae. This new species forms a polytomy at the crown with Pachyrhinosaurus canadensis and Pachyrhinosaurus perotorum, with Pachyrhinosaurus lakustai falling basal to that polytomy. The diagnostic features of this new species are an apomorphic, laterally curved Process 3 horns and a thick longitudinal ridge separating the supraorbital bosses. Another focus is investigating the ontogeny of Pachyrhinosaurus nasal bosses in a histological context. Previously, little work has been done on cranial histology in ceratopsians, focusing instead on potential integumentary structures, the parietals of Triceratops, and how surface texture relates to underlying histological structures. An ontogenetic series is established for the nasal bosses of Pachyrhinosaurus at both relative (subadult versus adult) and fine scale (Stages 1-5). It was demonstrated that histology alone can indicate relative ontogenetic level, but not stages of a finer scale. Through Pachyrhinosaurus ontogeny the nasal boss undergoes increased vascularity and secondary remodeling with a reduction in osteocyte lacunar density.