Bio-Energy Technologies Review
Total Page:16
File Type:pdf, Size:1020Kb
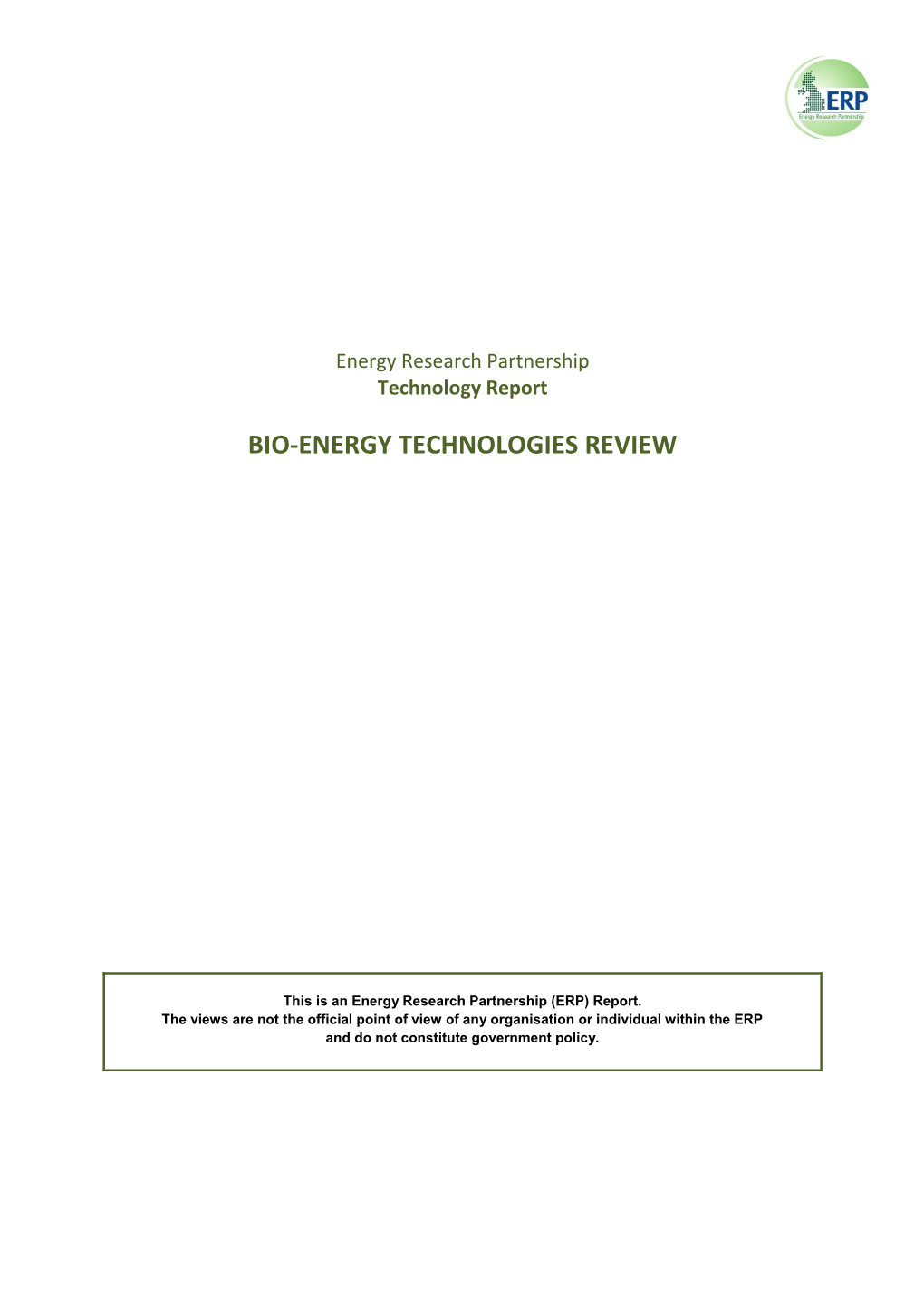
Load more
Recommended publications
-
IB Process Plant Study Page 2 of 107
Industrial Biotechnology Process Plant Study March 2015 A report for: The Biotechnology and Biological Sciences Research Council (BBSRC), The Engineering and Physical Sciences Research Council (EPSRC), Innovate UK and The Industrial Biotechnology Leadership Forum (IBLF). Authors: David Turley1, Adrian Higson1, Michael Goldsworthy1, Steve Martin2, David Hough2, Davide De Maio1 1 NNFCC 2 Inspire Biotech Approval for release: Adrian Higson Disclaimer While NNFCC and Inspire biotech considers that the information and opinions given in this work are sound, all parties must rely on their own skill and judgement when making use of it. NNFCC will not assume any liability to anyone for any loss or damage arising out of the provision of this report. NNFCC NNFCC is a leading international consultancy with expertise on the conversion of biomass to bioenergy, biofuels and bio-based products. NNFCC, Biocentre, Phone: +44 (0)1904 435182 York Science Park, Fax: +44 (0)1904 435345 Innovation Way, E: [email protected] Heslington, York, Web: www.nnfcc.co.uk YO10 5DG. IB Process Plant Study Page 2 of 107 Acknowledgement NNFCC wishes to acknowledge the input of the many stakeholders who provided information on the pilot scale equipment present in their respective facilities and more specifically the following stakeholders who gave of their time and experience, either in the workshop, or in one-to-one discussions with the project team. We would like to thank all for their valued input. Sohail Ali Plymouth Marine Laboratory Mike Allen Plymouth Marine Laboratory -
Long-Term Prospects for Northwest European Refining
LONG-TERM PROSPECTS FOR NORTHWEST EUROPEAN REFINING ASYMMETRIC CHANGE: A LOOMING GOVERNMENT DILEMMA? ROBBERT VAN DEN BERGH MICHIEL NIVARD MAURITS KREIJKES CIEP PAPER 2016 | 01 CIEP is affiliated to the Netherlands Institute of International Relations ‘Clingendael’. CIEP acts as an independent forum for governments, non-governmental organizations, the private sector, media, politicians and all others interested in changes and developments in the energy sector. CIEP organizes lectures, seminars, conferences and roundtable discussions. In addition, CIEP members of staff lecture in a variety of courses and training programmes. CIEP’s research, training and activities focus on two themes: • European energy market developments and policy-making; • Geopolitics of energy policy-making and energy markets CIEP is endorsed by the Dutch Ministry of Economic Affairs, the Dutch Ministry of Foreign Affairs, the Dutch Ministry of Infrastructure and the Environment, BP Europe SE- BP Nederland, Coöperatieve Centrale Raiffeisen-Boerenleenbank B.A. ('Rabobank'), Delta N.V., ENGIE Energie Nederland N.V., ENGIE E&P Nederland B.V., Eneco Holding N.V., EBN B.V., Essent N.V., Esso Nederland B.V., GasTerra B.V., N.V. Nederlandse Gasunie, Heerema Marine Contractors Nederland B.V., ING Commercial Banking, Nederlandse Aardolie Maatschappij B.V., N.V. NUON Energy, TenneT TSO B.V., Oranje-Nassau Energie B.V., Havenbedrijf Rotterdam N.V., Shell Nederland B.V., TAQA Energy B.V.,Total E&P Nederland B.V., Koninklijke Vopak N.V. and Wintershall Nederland B.V. CIEP Energy -
Planning Implications of Renewable and Low Carbon Energy
Practice Guidance Planning Implications of Renewable and Low Carbon Energy February 2011 Cover image courtesy of Thermal Earth Ltd Planning Divison Welsh Assembly Government Cardiff CF10 3NQ E-mail: [email protected] Planning web site - www.wales.gov.uk/planning ISBN 978 0 7504 6039 2 © Crown copyright 2011 WAG10-11462 F7131011 Table of Contents 1. Introduction 1 2. Renewable and Low Carbon Energy Technologies 10 3. Wind Energy 13 4. Biomass 27 5. Biomass – Anaerobic Digestion 43 6. Biofuels 49 7. Hydropower 55 8. Solar 62 9. Ground, Water and Air Source Heat Pumps 68 10. Geothermal 73 11. Fuel Cells 77 12. Combined Heat and Power/Combined Cooling Heat and Power 82 13. District Heating 86 14. Waste Heat 90 15. Cumulative Effects 96 16. Climate Change Effects 97 17. Financial Opportunities and Barriers 102 18. Community involvement and benefits 106 19. Renewable and Low Carbon developments in designated areas and 114 sites 20. Influencing planning decisions 124 Appendices Appendix 1: References 133 Appendix 2: Glossary 135 Appendix 3: Matrices – Potential Impacts of Renewable Energy Technologies (see separate spreadsheet) 3 Practice Guidance – Planning Implications of Renewable and Low Carbon Energy List of Abbreviations AAP Area Action Plan LAPC Local Air Pollution Control AD Anaerobic Digestion LDP Local Development Plan Area of Outstanding Natural AONB LPA Local Planning Authority Beauty Building Research Local Development BREEAM Establishment Environmental LDF Framework Assessment Method CAA Civil Aviation Authority -
Long-Term Experiments
ROTHAMSTED LONG-TERM EXPERIMENTS Guide to the Classical and other Long-term experiments, Datasets and Sample Archive Edited by A. J. Macdonald Contributors Andy Macdonald, Paul Poulton, Ian Clark, Tony Scott, Margaret Glendining, Sarah Perryman, Jonathan Storkey, James Bell, Ian Shield, Vanessa McMillan and Jane Hawkins. Rothamsted Research Harpenden, Herts, AL5 2JQ, UK Tel +44 (0) 1582 763133 Fax +44 (0) 1582 760981 www.rothamsted.ac.uk 1 CONTENTS INTRODUCTION 4 THE CLASSICAL EXPERIMENTS 7 Broadbalk Winter Wheat 7 Broadbalk and Geescroft Wildernesses 19 Park Grass 20 Hoosfield Spring Barley 31 Exhaustion Land 34 Garden Clover 36 OTHER LONG-TERM EXPERIMENTS 37 At Rothamsted 37 At Woburn 39 RESERVED AND DISCONTINUED EXPERIMENTS 41 Barnfield 41 Hoosfield Alternate Wheat and Fallow 41 Woburn Market Garden 42 Agdell 42 The Woburn Intensive Cereals Experiments 43 Saxmundham, Rotations I & II 43 Amounts of Straw and Continuous Maize Experiments 44 (Rothamsted and Woburn) METEOROLOGICAL DATA 45 LONG-TERM EXPERIMENTS AS A RESOURCE 45 THE ROTHAMSTED SAMPLE ARCHIVE 47 ELECTRONIC ROTHAMSTED ARCHIVE (e-RA) 49 THE ROTHAMSTED INSECT SURVEY (RIS) 50 UK ENVIRONMENTAL CHANGE NETWORK (ECN) 52 NORTH WYKE FARM PLATFORM 53 MAP OF ROTHAMSTED FARM 28 REFERENCES 56 The Rothamsted Long-term Experiments are supported by the UK Biotechnology and Biological Sciences Research Council Front cover under the National Capabilities programme Broadbalk from the air, 2015 2018 © Rothamsted Research grant (BBS/E/C/000J0300), and by the Back cover ISBN 978-1-9996750-0-4 (Print) Lawes Agricultural Trust. Park Grass from the air, 2015 ISBN 978-1-9996750-1-1 (Online) 2 FOREWORD It is a testament to the foresight and Managing and documenting these experiments commitment of Sir John Lawes and Sir Henry and their associated data and archives is Gilbert, as well as others who have come after not a trivial task. -
Revised Edition 2019 ACKNOWLEDGEMENTS
Revised Edition 2019 ACKNOWLEDGEMENTS Written and researched by: Ian Fitzpatrick, Richard Young and Robert Barbour with Megan Perry, Emma Rose and Aron Marshall. We would like to thank: Kath Dalmeny Adele Jones Christopher Stopes David Gould Stuart Meikle Marie Christine Mehrens Anil Graves Dominic Moran Thomas Harttung Jules Pretty Patrick Holden Hannah Steenbergen for helpful comments on draft versions or sections of the report. All interpretation, opinion and error is the responsibility of the authors alone. Designed by: Alan Carmody, Midas Design Consultants Ltd. and Blue Moon Creative Production Coordinator: Hannah Steenbergen Printed by Vale Press Ltd. First published November 2017 Revised and corrected July 2019 We would like to thank the following organisations for their invaluable support for our work on True Cost Accounting, as well as the Brunswick Group, who kindly hosted our report launch in November 2017: THE HIDDEN COST OF UK FOOD FOREWORD 3 PREFACE TO THE 2019 EDITION 5 PREFACE 7 EXECUTIVE SUMMARY 8 Hidden costs in 2015 ............................................................................................................................................8 Challenges to be overcome .............................................................................................................................10 Addressing the challenges ..............................................................................................................................10 The purpose of this report ...............................................................................................................................10 -
Agrochemicals - the Silent Killers Case Histories
Case histories Agrochemicals - the Silent Killers Rosemary Mason MB, ChB, FRCA and Palle Uhd Jepsen former Conservation Adviser to the Danish Forest and Nature Agency JUSTIFICATION The purpose of this document is to highlight the problems of the current and future use of agrochemical products, using a series of case studies. Have we forgotten Rachel Carson’s Silent Spring from 1962? Many of these chemicals are far more toxic (and persistent) than DDT. They are the silent destroyers of human health and the environment. CONTENTS CASE HISTORIES 2 Honeybees 2 Bumblebees 3 Super-weeds 5 The controversial BBC Countryfile programme 6 Why are the European authorities determined to get GM crops into Europe? 7 EFSA has recently given positive opinions on old herbicides at the request of industry 8 Another GM, herbicide tolerant seed in the pipeline 9 What is the role of the Commissioner of the Health and Consumers Directorate? 9 The effects of GM crops on humans in Latin America 10 Glyphosate-Based Herbicides Produce Teratogenic Effects on Vertebrates by Impairing Retinoic Acid Signaling 13 Danish farmers report side effects with GM Soya fed to pigs 14 Desiccation of crops with glyphosate to dry them 15 Scientists complain that the EC has ignored independent scientific advice about Roundup® 15 RMS (DAR) studies on glyphosate 16 Other EFSA reasoned opinions for modification of MRLs in food 17 Lack of ecological knowledge from industry and governments 17 Humans are bearing the brunt of these genotoxic chemicals and will do so even more 18 The Faroes Statement: Human Health Effects of Developmental Exposure to Chemicals in Our Environment 2007 19 The Permanent Peoples’ Tribunal 19 Peoples’ Submission 19 The Verdict 23 Summary of Verdict by members of the Jury 23 1 Summary of complaints to the Ombudsman 1360/2012/BEH about the EC and EFSA CASE HISTORIES Honeybees Dead queens and workers. -
Insect Biochemistry and Molecular Biology 48 (2014) 51E62
Insect Biochemistry and Molecular Biology 48 (2014) 51e62 Contents lists available at ScienceDirect Insect Biochemistry and Molecular Biology journal homepage: www.elsevier.com/locate/ibmb Identification of pheromone components and their binding affinity to the odorant binding protein CcapOBP83a-2 of the Mediterranean fruit fly, Ceratitis capitata P. Siciliano a,b, X.L. He a, C. Woodcock a, J.A. Pickett a, L.M. Field a, M.A. Birkett a, B. Kalinova c, L.M. Gomulski b, F. Scolari b, G. Gasperi b, A.R. Malacrida b, J.J. Zhou a,* a Department of Biological Chemistry and Crop Protection, Rothamsted Research, Harpenden, Herts. AL5 2JQ, United Kingdom b Dipartimento di Biologia e Biotecnologie, Università di Pavia, Via Ferrata 9, 27100 Pavia, Italia c Institute of Organic Chemistry and Biochemistry of the AS CR, v.v.i., Flemingovo nám. 2, CZ-166 10 Prague 6, Czech Republic article info abstract Article history: The Mediterranean fruit fly (or medfly), Ceratitis capitata (Wiedemann; Diptera: Tephritidae), is a serious Received 30 July 2013 pest of agriculture worldwide, displaying a very wide larval host range with more than 250 different Received in revised form species of fruit and vegetables. Olfaction plays a key role in the invasive potential of this species. Un- 17 February 2014 fortunately, the pheromone communication system of the medfly is complex and still not well estab- Accepted 18 February 2014 lished. In this study, we report the isolation of chemicals emitted by sexually mature individuals during the “calling” period and the electrophysiological responses that these compounds elicit on the antennae Keywords: fl fl of male and female ies. -
Lignocellulosic Feedstock in the UK
Lignocellulosic feedstock in the UK November 2014 A report for the Lignocellulosic Biorefinery Network Authors: Davide Di Maio and David Turley Reviewer: Lucy Hopwood Disclaimer While NNFCC considers that the information and opinions given in this work are sound, all parties must rely on their own skill and judgement when making use of it NNFCC will not assume any liability to anyone for any loss or damage arising out of the provision of this report. NNFCC NNFCC is a leading international consultancy with expertise on the conversion of biomass to bioenergy, biofuels and bio-based products. NNFCC, Biocentre, Phone: +44 (0)1904 435182 York Science Park, Fax: +44 (0)1904 435345 Innovation Way, E: [email protected] Heslington, York, Web: www.nnfcc.co.uk YO10 5DG. 2 Executive Summary NNFCC was commissioned by the Lignocellulosic Biorefinery Network (LBNet) to survey the potential availability of existing domestic lignocellulosic non-food crop feedstock wastes and residues to support the development of UK biorefineries. The objective was to assess the current availability of domestic crop and forest residues; dedicated biomass crops; green waste and waste from the paper industry. Current competing uses for these materials were identified and the potential to expand the resource examined. Impacts of regional and temporal variability were considered and data on costs and composition were collated. The analysis highlighted that the UK has nearly 16 million tons of biomass waste arising from the feedstocks studied. The greatest contributions to this total are from green waste, agricultural straw and a significant amount of waste paper that is currently collected but not recycled in the UK. -
Large Industrial Users of Energy Biomass
12 September 2013 Authors Esa Vakkilainen, Katja Kuparinen, Jussi Heinimö Lappeenranta University of Technology www.lut.fi Published in 12th September 2013 Cover pictures by Foster Wheeler, Esa Vakkilainen and Andritz Disclaimer This report was written for IEA Bioenergy Task 40. The sole responsibility for the content of this publication lies with the authors. It does not necessarily reflect the opinion of the IEA or the members of the IEA Bioenergy Implementing agreement. IEA Bioenergy Task 40 has reviewed and approved this report, but is not responsible for any use that may be made of the information or opinions contained therein. 12 September 2013 Terms Bioenergy Bioenergy refers to energy derived from biofuel. Biomass Refers to the biodegradable fraction of products, waste and residues from agriculture (including vegetal and animal substances), forestry and related industries, as well as the biodegradable fraction of industrial and municipal waste. Biofuel (=biomass fuel) Fuel produced directly or indirectly from biomass. The fuel may have undergone mechanical, chemical or biological processing or conversion or it may have had a previous use. Biofuel refers to solid, gaseous and liquid biomass-derived fuels. Energy biomass Refers to biomass that is utilised for energy purposes. Conversion factors Following conversion factors are used in this report: Wood pellets lower heating value 17.0 MJ/kg Ethanol lower heating value 21.2 MJ/l density 0.8 kg/l Biodiesel lower heating value 37.3 MJ/kg density 0.88 kg/l Charcoal lower heating value 22.0 MJ/kg Pyrolysis oil lower heating value 17.0 MJ/kg density 1.2 kg/l 12 September 2013 Executive summary The markets of energy biomass are developing rapidly and becoming more international. -
Saturday 18 July 2009
Contents House of Commons • Noticeboard ..........................................................................................................1 • The Week Ahead..................................................................................................2 • Order of Oral Questions .......................................................................................3 Weekly Business Information • Business of the House of Commons 13 – 17 July 2009........................................5 Bulletin • Written Ministerial Statements.............................................................................7 • Forthcoming Business of the House of Commons 20 July – 16 October 2009..10 • Forthcoming Business of the House of Lords 20 July – 16 October 2009 .........18 Editor: Kevin Williams Legislation House of Commons Public Legislation Information Office • Public Bills before Parliament 2008/09..............................................................20 London • Bills – Presentation, Publication and Royal Assent............................................30 SW1A 2TT • Public and General Acts 2008/09 .......................................................................31 www.parliament.uk • Draft Bills under consideration or published during 2008/09 Session ...............32 Tel : 020 7219 4272 Private Legislation Fax : 020 7219 5839 • Private Bills before Parliament 2008/09.............................................................33 [email protected] Delegated Legislation • Statutory Instruments .........................................................................................36 -
2014 Reference Document Reference Document
AREVA supplies high added-value products and services to support the operation of the global nuclear fl eet. The company is present throughout the entire nuclear cycle, from uranium mining to used fuel recycling, including nuclear reactor design and operating services. AREVA is recognized by utilities around the world for its expertise, its skills in cutting-edge technologies and its dedication to the highest level of safety. Through partnerships, the company is active in the renewable energy sector. AREVA’s 44,000 employees are helping build tomorrow’s energy model: supplying ever safer, cleaner and more economical energy to the greatest number of people. www.areva.com 2014 2014 Reference document Reference document FINANCIAL COMMUNICATIONS DEPARTMENT Tour AREVA - 1, place Jean Millier - 92400 Courbevoie – France - Te l.: 33 1 34 96 06 47 - Fax: +33 1 34 96 99 00 Energy is our future, don’t waste it! Reference document REFERENCE 20 DOCUMENT 14 This Reference Document was fi led with the Autorité des marchés fi nanciers (AMF, the French fi nancial market authority) on March 31, 2015 , in accordance with article 212-13 of its general regulations. It may be used in support of a fi nancial transaction if it is accompanied by an off ering circular si gned by the AMF. This document was prepared by the issuer and is binding on those signing it. This is a free translation into English of the AREVA group’s Reference Document for 2014, which is issued in the French language, and is provided solely for the convenience of English speaking readers. -
Annex D Major Events in the Energy Industry
Annex D Major events in the Energy Industry 2017 Electricity The foundation stone for the new ElecLink electricity connection between Britain and France was laid in February 2017. The interconnector will run through the Channel Tunnel between Sellindge in the UK and Les Mandarins in France, and will provide 1000MW of electricity, enough capacity to power up to 2 million homes. Energy Efficiency Homes across Great Britain will get extra support to make their homes cheaper and easier to keep warm thanks to reforms that came into force in April 2017. Changes to the Energy Company Obligation (ECO) will make sure energy companies give support to people struggling to meet their heating bills, with plans announced to extend the scheme from April 2017 to September 2018. Smart Meters A Smart Meters bill was included in the Queen’s speech in June 2017 to allow the Government to continue to oversee the successful completion of the rollout of smart meters and protect consumers, leading to £5.7 billion of net benefits to Britain. 2016 Energy Policy The Energy Bill received Royal Assent in May 2016. In summary the Bill: Creates the framework to formally establish the Oil and Gas Authority (OGA) as an independent regulator, taking the form of a government company, so that it can act with greater flexibility and independence. It gives the OGA new powers including: access to external meetings; data acquisition and retention; dispute resolution; and sanctions. It also enables the transfer of the Secretary of State of the Department for Business, Energy and Industrial Strategy (BEIS) existing regulatory powers in respect of oil and gas to the OGA.