Introduction to Flight Test Engineering (Introduction Aux Techniques Des Essais En Vol)
Total Page:16
File Type:pdf, Size:1020Kb
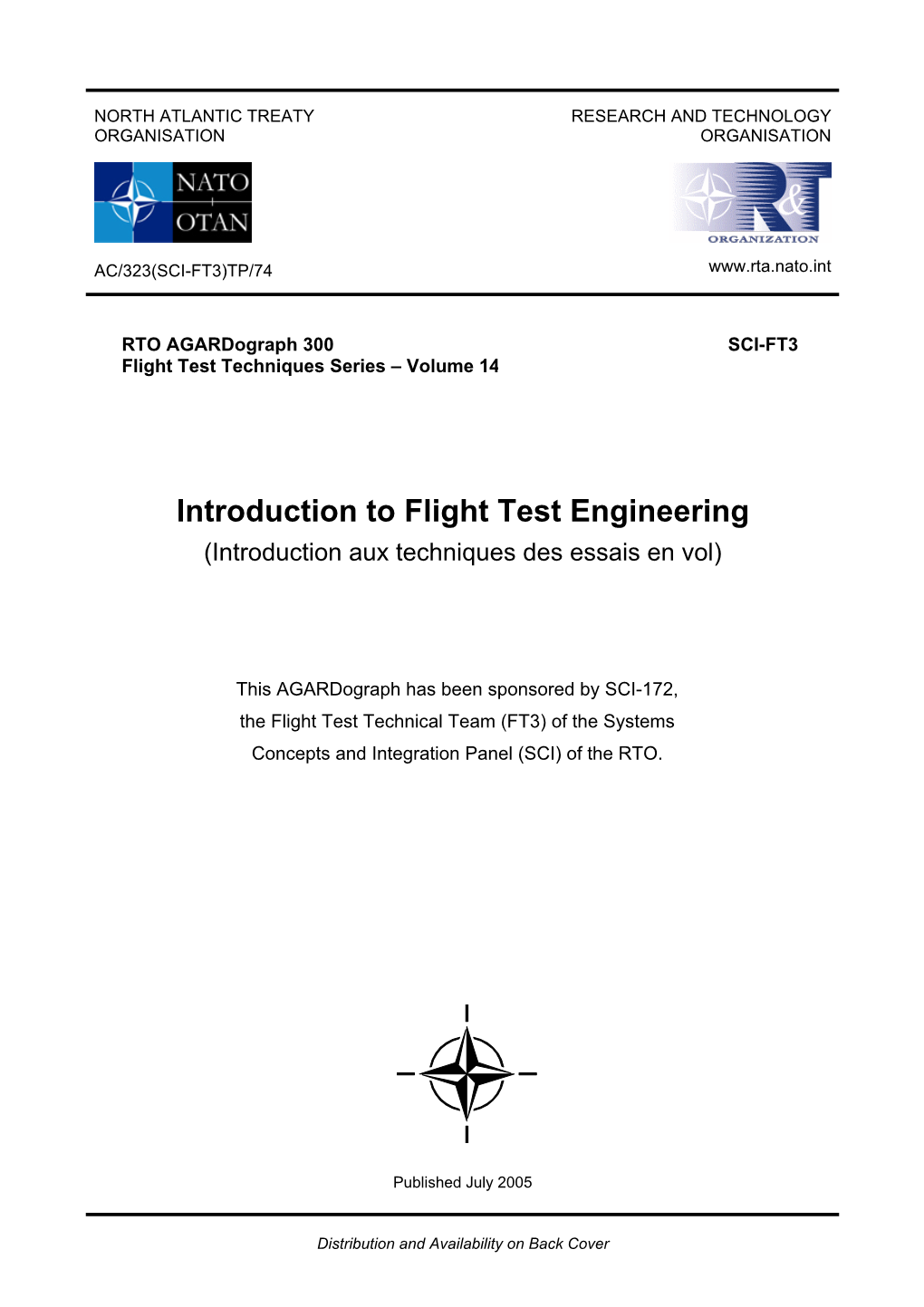
Load more
Recommended publications
-
Validation of Wind Tunnel Test and Cfd Techniques for Retro-Propulsion (Retpro): Overview on a Project Within the Future Launchers Preparatory Programme (Flpp)
VALIDATION OF WIND TUNNEL TEST AND CFD TECHNIQUES FOR RETRO-PROPULSION (RETPRO): OVERVIEW ON A PROJECT WITHIN THE FUTURE LAUNCHERS PREPARATORY PROGRAMME (FLPP) D. Kirchheck, A. Marwege, J. Klevanski, J. Riehmer, A. Gulhan¨ German Aerospace Center (DLR) Supersonic and Hypersonic Technologies Department Cologne, Germany S. Karl O. Gloth German Aerospace Center (DLR) enGits GmbH Spacecraft Department Todtnau, Germany Gottingen,¨ Germany ABSTRACT and landing (VTVL) spacecraft, assisted by retro-propulsion. Up to now, in Europe, knowledge and expertise in that field, The RETPRO project is a 2-years activity, led by the Ger- though constantly growing, is still limited. Systematic stud- man Aerospace Center (DLR) in the frame of ESA’s Future ies were conducted to compare concepts for possible future Launchers Preparatory Program (FLPP), to close the gap of European launchers [2, 3], and activities on detailed inves- knowledge on aerodynamics and aero-thermodynamics of tigations of system components of VTVL re-usable launch retro-propulsion assisted landings for future concepts in Eu- vehicles (RLV) recently started in the RETALT project [4, 5]. rope. The paper gives an overview on the goals, strategy, and Nevertheless, validated knowledge on the aerodynamic current status of the project, aiming for the validation of inno- and aerothermal characteristics of such vehicles is still lim- vative WTT and CFD tools for retro-propulsion applications. ited to a small amount of experimental and numerical inves- Index Terms— RETPRO, retro-propulsion, launcher tigations mostly on lower altitude VTVL trajectories, e. g. aero-thermodynamics, wind tunnel testing, CFD validation within the CALLISTO project [6, 7, 8]. Other studies were conducted to analyze the aerothermodynamics of a simplified generic Falcon 9 geometry during its re-entry and landing 1. -
Reusable Launch Vehicle Technology Program{
Acta Astronautica Vol. 41, No. 11, pp. 777±790, 1997 # 1998 Published by Elsevier Science Ltd. All rights reserved Printed in Great Britain PII: S0094-5765(97)00197-5 0094-5765/98 $19.00 + 0.00 REUSABLE LAUNCH VEHICLE TECHNOLOGY PROGRAM{ DELMA C. FREEMAN{ JR. and THEODORE A. TALAY} NASA Langley Research Center, Hampton, Virginia 23681-0001, USA R. EUGENE AUSTIN} NASA Marshall Space Flight Center, Marshall Space Flight Center, Alabama 35812-1000, USA (Received 25 April 1997) AbstractÐIndustry/NASA reusable launch vehicle (RLV) technology program eorts are underway to design, test, and develop technologies and concepts for viable commercial launch systems that also satisfy national needs at acceptable recurring costs. Signi®cant progress has been made in understanding the technical challenges of fully reusable launch systems and the accompanying management and oper- ational approaches for achieving a low-cost program. This paper reviews the current status of the RLV technology program including the DC-XA, X-33 and X-34 ¯ight systems and associated technology programs. It addresses the speci®c technologies being tested that address the technical and operability challenges of reusable launch systems including reusable cryogenic propellant tanks, composite structures, thermal protection systems, improved propul- sion, and subsystem operability enhancements. The recently concluded DC-XA test program demon- strated some of these technologies in ground and ¯ight tests. Contracts were awarded recently for both the X-33 and X-34 ¯ight demonstrator systems. The Orbital Sciences Corporation X-34 ¯ight test ve- hicle will demonstrate an air-launched reusable vehicle capable of ¯ight to speeds of Mach 8. -
A Review of Current Research in Subscale Flight Testing and Analysis of Its Main Practical Challenges
aerospace Article A Review of Current Research in Subscale Flight Testing and Analysis of Its Main Practical Challenges Alejandro Sobron * , David Lundström and Petter Krus Department of Management and Engineering, Division of Fluid and Mechatronic Systems, Linköping University, SE-58183 Linköping, Sweden; [email protected] (D.L.); [email protected] (P.K.) * Correspondence: [email protected]; Tel.: +46-1328-1893 Abstract: Testing of untethered subscale models, often referred to as subscale flight testing, has traditionally had a relatively minor, yet relevant use in aeronautical research and development. As recent advances in electronics, rapid prototyping and unmanned-vehicle technologies expand its capabilities and lower its cost, this experimental method is seeing growing interest across academia and the industry. However, subscale models cannot meet all similarity conditions required for simulating full-scale flight. This leads to a variety of approaches to scaling and to other alternative applications. Through a literature review and analysis of different scaling strategies, this study presents an overall picture of how subscale flight testing has been used in recent years and synthesises its main issues and practical limitations. Results show that, while the estimation of full-scale characteristics is still an interesting application within certain flight conditions, subscale models are progressively taking a broader role as low-cost technology-testing platforms with relaxed similarity constraints. Different approaches to tackle the identified practical challenges, implemented both by the authors and by other organisations, are discussed and evaluated through flight experiments. Citation: Sobron, A.; Lundström, D.; Keywords: subscale flight testing; similarity; scale model; remotely piloted aircraft; demonstration; Krus, P. -
+ Part 10: Test and Evaluation
10. Test and Evaluation 10.1 Approach Architecture Design, Development, Test, and Evaluation (DDT&E) schedule, costs, and risk are highly dependent on the integrated test and evaluation approach for each of the major elements. As a part of the Exploration Systems Architecture Study (ESAS), a top-level test and evaluation plan, including individual flight test objectives, was developed and is summarized in this section. The test and evaluation plan described here is derived from the Apollo Flight Test Program of the 1960s. A more detailed test and evaluation plan will be based on detailed verification requirements and objectives documented in specifications and verification plans. In order to support schedule, cost, and risk assessments for the reference ESAS architecture, an integrated test and evaluation plan was developed to identify the number and type of major test articles (flight and ground) and the timing and objectives of each major flight test, including facilities and equipment required to support those tests. This initial plan is based on the Apollo Program and the ESAS Ground Rules and Assumptions (GR&As)—including the human- rating requirements from NASA Procedural Requirements (NPR) 8705.2A, Human-Rating Requirements for Space Systems. 10. Test and Evaluation 645 10.2 Ground Rules and Assumptions ESAS GR&As establish the initial set of key constraints to testing. Although all ESAS GR&As are considered, the specific ones listed below are particularly significant, as they deal with schedule and testing/qualification assumptions. • The crew launch system shall facilitate crew survival using abort and escape. There will be three all-up tests of the Launch Abort System (LAS). -
Propulsion and Flight Controls Integration for the Blended Wing Body Aircraft
Cranfield University Naveed ur Rahman Propulsion and Flight Controls Integration for the Blended Wing Body Aircraft School of Engineering PhD Thesis Cranfield University Department of Aerospace Sciences School of Engineering PhD Thesis Academic Year 2008-09 Naveed ur Rahman Propulsion and Flight Controls Integration for the Blended Wing Body Aircraft Supervisor: Dr James F. Whidborne May 2009 c Cranfield University 2009. All rights reserved. No part of this publication may be reproduced without the written permission of the copyright owner. Abstract The Blended Wing Body (BWB) aircraft offers a number of aerodynamic perfor- mance advantages when compared with conventional configurations. However, while operating at low airspeeds with nominal static margins, the controls on the BWB aircraft begin to saturate and the dynamic performance gets sluggish. Augmenta- tion of aerodynamic controls with the propulsion system is therefore considered in this research. Two aspects were of interest, namely thrust vectoring (TVC) and flap blowing. An aerodynamic model for the BWB aircraft with blown flap effects was formulated using empirical and vortex lattice methods and then integrated with a three spool Trent 500 turbofan engine model. The objectives were to estimate the effect of vectored thrust and engine bleed on its performance and to ascertain the corresponding gains in aerodynamic control effectiveness. To enhance control effectiveness, both internally and external blown flaps were sim- ulated. For a full span internally blown flap (IBF) arrangement using IPC flow, the amount of bleed mass flow and consequently the achievable blowing coefficients are limited. For IBF, the pitch control effectiveness was shown to increase by 18% at low airspeeds. -
Flight Engineer Knowledge Test Guide
AC 63-1 FLIGHT ENGINEER KNOWLEDGE TEST GUIDE U.S. Department of Transportation Federal Aviation Administration 1 FLIGHT ENGINEER KNOWLEDGE TEST GUIDE 1995 U.S. DEPARTMENT OF TRANSPORTATION FEDERAL AVIATION ADMINISTRATION Flight Standards Service 2 PREFACE The Flight Standards Service of the Federal Aviation Administration (FAA) has developed this guide to help applicants meet the knowledge requirements for the computer administered tests for flight engineer turbojet, turboprop, and reciprocating class certification. This guide contains information about the knowledge test eligibility requirements, test descriptions, testing and retesting procedures, and sample test questions with answers. As a convenience to the applicant, the eligibility requirements for the oral and flight tests are included. Appendix 1 provides a list of reference materials and subject matter knowledge codes, and computer testing designees. Changes to the subject matter knowledge code list will be published as a separate advisory circular. The sample questions and answers in this guide are predicated on Federal Aviation Regulations (FAR's) and references that were current at the time of publication. Questions and answers in the computer administered knowledge tests are updated when changes are made to these reference materials. The flight engineer test question bank and subject matter knowledge code list for all airmen certificates and ratings, with changes, may be obtained by computer access from FedWorld at (703) 321-3339. This bulletin board service is provided by the U.S. Department of Commerce, 24 hours a day, 7 days per week. For technical assistance regarding computer requirements for this service, contact the FedWorld help desk at (703) 487-4608 from 7:30 a.m. -
SETP/SFTE Flight Test Safety Workshop Wednesday 17Th
Conference & Events www.aerosociety.com/conference SETP/SFTE Flight Test Safety Workshop Wednesday 17th – Thursday 18th October 2007 No. 4 Hamilton Place, London, UK PROGRAMME Tuesday 16th October 19.00 Welcome Reception Wednesday 17th October 08.00 Registration and Coffee 08.30 Welcome & Introduction Thomas E. Roberts, RDT&E Safety Manager, Naval Test Wing Atlantic & Flight Test Safety Committee Dennis Morley, Chief Flight Test Engineer, BAE Systems Maurice Girard, Senior Engineering Test Pilot, Cessna Engineering Flight Test SESSION 1: CHAIRMAN: Rod Huete, Chairman, Federal Aviation Authority 08.45 Global War on Error for the Test Team Pat Daily, Executive Vice President, Convergent-Knowledge & Rogers Smith, National Test Pilots School 10.45 Tea 11.15 Incorporating a Safety Management System into Flight Test and Research Operations Tim Leslie, National Research Council 11.50 How a Federal Civil Certification Agency Deals with Flight Test Risk Management Rod Huete, Chairman, Federal Aviation Authority 12.25 Panel Discussion 12.50 Lunch SESSION 2: CHAIRMAN: TBC 13.50 F-16 Block 60 Ejection Dan Levin & Billie Flynn, Experimental Test Pilot, Lockheed Martin 14.25 High Incidence Flight Testing Risk Management Terry Smith, BAE Systems 15:00 Protecting the Flight Test Program Dr. Guy Gratton, CEng FRAes MIMechE MSETP, Chairman, Association of Aerospace Universities Aircraft Operations and General Aviation Committee, Royal Aeronautical Society/T.C. Porteous, Positions tbc, British Microlight Aircraft Association 15.35 Coffee 16.10 Toward Improved Flight Test Planning and Data Analysis Options Dean Carico, United States Navy 16.45 A Comparison of the Hollywood Stunt Business and Aircraft Flight Testing Rusty Lowery, USN TPS 17.10 Panel Discussion 17.30 Close of Day One Proceedings 19.00 Dinner Royal Aeronautical Society, No.4 Hamilton Place, London W1J 7BQ, United Kingdom VAT Number 672 8668 84 Tel: +44 (0)20 7670 4345 Fax +44 (0)20 7670 4349 Email: [email protected] Registered Charity No. -
Unusual Attitudes and the Aerodynamics of Maneuvering Flight Author’S Note to Flightlab Students
Unusual Attitudes and the Aerodynamics of Maneuvering Flight Author’s Note to Flightlab Students The collection of documents assembled here, under the general title “Unusual Attitudes and the Aerodynamics of Maneuvering Flight,” covers a lot of ground. That’s because unusual-attitude training is the perfect occasion for aerodynamics training, and in turn depends on aerodynamics training for success. I don’t expect a pilot new to the subject to absorb everything here in one gulp. That’s not necessary; in fact, it would be beyond the call of duty for most—aspiring test pilots aside. But do give the contents a quick initial pass, if only to get the measure of what’s available and how it’s organized. Your flights will be more productive if you know where to go in the texts for additional background. Before we fly together, I suggest that you read the section called “Axes and Derivatives.” This will introduce you to the concept of the velocity vector and to the basic aircraft response modes. If you pick up a head of steam, go on to read “Two-Dimensional Aerodynamics.” This is mostly about how pressure patterns form over the surface of a wing during the generation of lift, and begins to suggest how changes in those patterns, visible to us through our wing tufts, affect control. If you catch any typos, or statements that you think are either unclear or simply preposterous, please let me know. Thanks. Bill Crawford ii Bill Crawford: WWW.FLIGHTLAB.NET Unusual Attitudes and the Aerodynamics of Maneuvering Flight © Flight Emergency & Advanced Maneuvers Training, Inc. -
Space Reporter's Handbook Mission Supplement
CBS News Space Reporter's Handbook - Mission Supplement! Page 1 The CBS News Space Reporter's Handbook Mission Supplement Shuttle Mission STS-124: Space Station Assembly Flight 1J Written and Edited By William G. Harwood Aerospace Writer/Consultant [email protected] CBS News!!! 7/4/11 Page 2 ! CBS News Space Reporter's Handbook - Mission Supplement Revision History Editor's Note Mission-specific sections of the Space Reporter's Handbook are posted as flight data becomes available. Readers should check the CBS News "Space Place" web site in the weeks before a launch to download the latest edition: http://www.cbsnews.com/network/news/space/current.html DATE RELEASE NOTES 05/28/08 Initial STS-124 release Introduction This document is an outgrowth of my original UPI Space Reporter's Handbook, prepared prior to STS-26 for United Press International and updated for several flights thereafter due to popular demand. The current version is prepared for CBS News. As with the original, the goal here is to provide useful information on U.S. and Russian space flights so reporters and producers will not be forced to rely on government or industry public affairs officers at times when it might be difficult to get timely responses. All of these data are available elsewhere, of course, but not necessarily in one place. The STS-124 version of the CBS News Space Reporter's Handbook was compiled from NASA news releases, JSC flight plans, the Shuttle Flight Data and In-Flight Anomaly List, NASA Public Affairs and the Flight Dynamics office (abort boundaries) at the Johnson Space Center in Houston. -
UAS FLIGHT TEST for SAFETY and for EFFICIENCY Seamus M
UAS FLIGHT TEST FOR SAFETY AND FOR EFFICIENCY Seamus M. McGovern, U.S. DOT National Transportation Systems Center, Cambridge, Massachusetts Abstract (e.g., SAE International technical standards and recommended practices) but also takes advantage of Manned aircraft that operate in the National competitive racing in order to evaluate new Airspace System (NAS) typically undergo technologies and materials (as it turns out, this may certification flight test to ensure they meet a have applicability to UASs as well with the advent of prescribed level of safety—dependent on their several organizations and sanctioning bodies category—before they are able to enter service [for including the European Rotor Sports Association and example, Federal Aviation Administration (FAA) the Drone Racing League). Other aviation-related advisory circular (AC) 25-7C is the flight-test guide tests and test formats not discussed here include the for certification of transport-category airplanes]. typically Department of Defense-focused With the integration of unmanned aircraft systems developmental test and evaluation; operational test (UAS) into the NAS, in the future some type of and evaluation; and research, development, test, and certification flight test may ultimately be required, evaluation structures. however, even lacking such a requirement UAS manufacturers can find value in flight testing UASs In terms of flight test, the military services have using familiar experimental and certification flight- their own criteria for evaluating their various aircraft. test procedures, the results of which can enhance the These requirements are often bound by contractual safety of the design, the safety of the operation, agreements between the service and the vendor, and/or the efficiency of the operation. -
General Flight Test Theory Applied to Aircraft Modifications
General Flight Test TheoryTUTORIAL Applied to Aircraft Modifications GENERAL FLIGHT TEST THEORY APPLIED TO AIRCRAFT MODIFICATIONS Lt Col Lionel D. Alford, Jr., USAF and Robert C. Knarr Any external aircraft modification has potentially far-reaching effects on the capability of the aircraft to succeed or fail in its mission. The authors take a systematic look at the effects that small changes can have upon the whole, with a series of examples that demonstrate why careful review of data or testing is often vital in the assessment of system modifications. new design aircraft program always external aircraft modification. The aircraft includes an instrumented test to design problems covered here represent Avalidate the analyses. But a modifi- the fundamental characteristics by which cation program may rely instead on pre- aircraft capability is judged. These design viously collected data for model valida- problems, when not properly analyzed and tion. Such a program must adequately tested (if required), have historically address the effects of the modification on resulted in significant degradation of air the aircraft and its mission. The user must worthiness. We define the subject area and judge these effects for their desirability— explain the importance of each problem especially when they degrade mission by discussing the rationale behind stan- capability. But, to be judged, they must dard design practices and air worthiness be fully understood. Reviewing historical and operational considerations for the fleet data or conducting a test are two ways to aircraft. Concrete examples illustrate each validate the data by which these effects case. Although only effects to the C–130 on aircraft capability are judged. -
PDF Download
September 2006 Volume V, Issue V www.boeing.com/frontiers ROARING SUCCESS The EA-18G Growler makes its first public appearance and takes its initial flight. Here’s why this new aircraft is important to Boeing and its customer. HEAVY DUTY 18 ‘Fab’ has role on special 747 COME TOGETHER 22 Japan Apache: Tech + people STAR POWER 26 Satellite business aims high www.boeing.com K Y M C ` F C & B & C F 60116-01 BCDEFG A Job Job : : Cust This ad, the third in a new series from the company’s portfolio of community ads, reinforces Boeing’s support of the arts, which help enrich and enlighten the lives of people worldwide. These ads are published in support of arts-related events. § JOB NUMBER: BOEG-0000-M1834 Version: A Frontiers CLIENT: Boeing PRODUCT: Corporate Communications DIVISION: None Date: 11/4/05 9:42 AM Colors: Process Cyan, Process Magenta, PDM: Scott Simpson File Name: m1834vA_R2_Opera_Frntr.indd Process Yellow, Process Black Editor: Pat Owens Media: ADV Mag Fonts: Helvetica (Light Oblique; Type 1), Agenda QC: Yanez Color Sp: 4C FRONTIERS (Nwp; Type 1) Images: M1834CT03_OperaFR_HR_r2.eps (305 ppi), Print Producer: Kim Nosalik Scale: 1=1 Boeing-FNF_rev_ad-StPg.eps Traffi c Supervisor: Kelly Riordan Bleed: 8.875 in x 11.25 in Headline: In the arts, we fi nd our wings. Art Director: Greg Auer Trim: 8.375 in x 10.75 in Notes: Bill to: m2039 Copywriter: None Safety: 7.375 in x 9.875 in ECD/GCD: Guy Bommarito Gutter: None Folds: None, None Account Exec: Leanne Mayer Output%: 100% Client: Boeing Art Buyer: Cameo Amato Legal: None Designer: Anne Perry Design Director: Wayne Carey Retoucher: Patrick McCabe Vendor: Schawk SEPTEMBER 2006 Volume V, Issue V ON THE COVER: In the skies above the St.