Evolutionary Dynamics of LINE-1 Retrotransposons Across the Eukaryotic Tree of Life
Total Page:16
File Type:pdf, Size:1020Kb
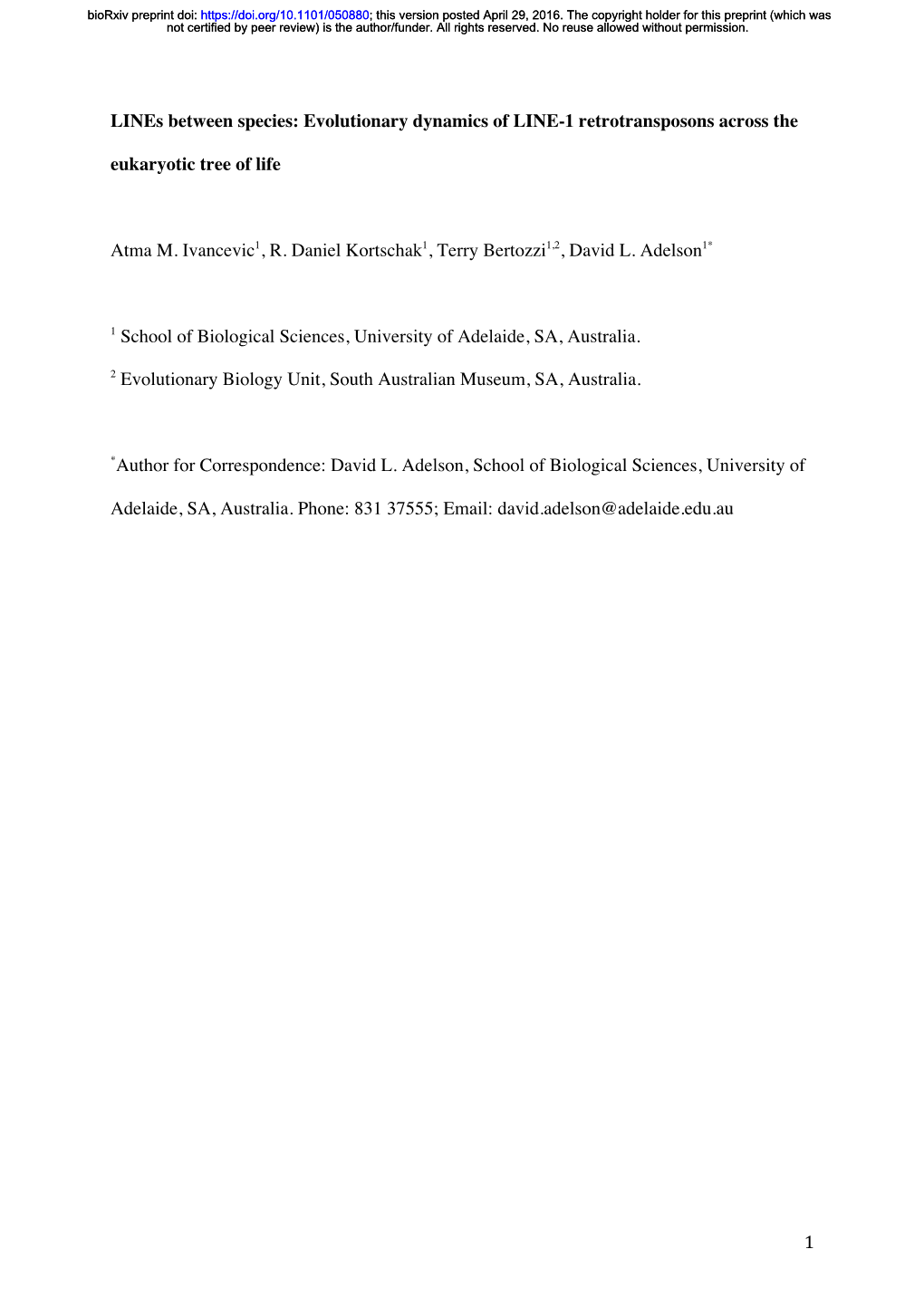
Load more
Recommended publications
-
Genome Skimming for Phylogenomics
Genome skimming for phylogenomics Steven Andrew Dodsworth School of Biological and Chemical Sciences, Queen Mary University of London, Mile End Road, London E1 4NS, UK. Submitted in partial fulfilment of the requirements of the degree of Doctor of Philosophy November 2015 1 Statement of originality I, Steven Andrew Dodsworth, confirm that the research included within this thesis is my own work or that where it has been carried out in collaboration with, or supported by others, that this is duly acknowledged and my contribution indicated. Previously published material is also acknowledged and a full list of publications is given in the Appendix. Details of collaboration and publications are given at the start of each chapter, as appropriate. I attest that I have exercised reasonable care to ensure that the work is original, and does not to the best of my knowledge break any UK law, infringe any third party’s copyright or other Intellectual Property Right, or contain any confidential material. I accept that the College has the right to use plagiarism detection software to check the electronic version of the thesis. I confirm that this thesis has not been previously submitted for the award of a degree by this or any other university. The copyright of this thesis rests with the author and no quotation from it or information derived from it may be published without the prior written consent of the author. Signature: Date: 16th November 2015 2 Frontispiece: Nicotiana burbidgeae Symon at Dalhousie Springs, South Australia. 2014. Photo: S. Dodsworth. 3 Acknowledgements Firstly, I would like to thank my PhD supervisors, Professor Andrew Leitch and Professor Mark Chase. -
Interfering with Retrotransposition by Two Types of CRISPR Effectors
Zhang et al. Cell Discovery (2020) 6:30 Cell Discovery https://doi.org/10.1038/s41421-020-0164-0 www.nature.com/celldisc ARTICLE Open Access Interfering with retrotransposition by two types of CRISPR effectors: Cas12a and Cas13a Niubing Zhang1,2, Xinyun Jing1, Yuanhua Liu3, Minjie Chen1,2, Xianfeng Zhu2, Jing Jiang2, Hongbing Wang4, Xuan Li1 and Pei Hao3 Abstract CRISPRs are a promising tool being explored in combating exogenous retroviral pathogens and in disabling endogenous retroviruses for organ transplantation. The Cas12a and Cas13a systems offer novel mechanisms of CRISPR actions that have not been evaluated for retrovirus interference. Particularly, a latest study revealed that the activated Cas13a provided bacterial hosts with a “passive protection” mechanism to defend against DNA phage infection by inducing cell growth arrest in infected cells, which is especially significant as it endows Cas13a, a RNA-targeting CRISPR effector, with mount defense against both RNA and DNA invaders. Here, by refitting long terminal repeat retrotransposon Tf1 as a model system, which shares common features with retrovirus regarding their replication mechanism and life cycle, we repurposed CRISPR-Cas12a and -Cas13a to interfere with Tf1 retrotransposition, and evaluated their different mechanisms of action. Cas12a exhibited strong inhibition on retrotransposition, allowing marginal Tf1 transposition that was likely the result of a lasting pool of Tf1 RNA/cDNA intermediates protected within virus-like particles. The residual activities, however, were completely eliminated with new constructs for persistent crRNA targeting. On the other hand, targeting Cas13a to Tf1 RNA intermediates significantly inhibited Tf1 retrotransposition. However, unlike in bacterial hosts, the sustained activation of Cas13a by Tf1 transcripts did not cause cell growth arrest in S. -
A Species-Specific Retrotransposon Drives a Conserved Cdk2ap1 Isoform Essential for Preimplantation Development
bioRxiv preprint doi: https://doi.org/10.1101/2021.03.24.436683; this version posted March 25, 2021. The copyright holder for this preprint (which was not certified by peer review) is the author/funder. All rights reserved. No reuse allowed without permission. Title: A species-specific retrotransposon drives a conserved Cdk2ap1 isoform essential for preimplantation development Authors: Andrew Modzelewski1†, Wanqing Shao2†, Jingqi Chen1, Angus Lee1, Xin Qi1, Mackenzie Noon1, Kristy Tjokro1, Gabriele Sales3, Anne Biton4, Terence Speed5, Zhenyu Xuan6, Ting Wang2#, Davide Risso3# and Lin He1# Affiliations: 1 Division of Cellular and Developmental Biology, MCB department, University of California at Berkeley, Berkeley, CA, USA. 2 Department of Genetics, Washington University School of Medicine, St. Louis, Missouri, USA 3 Department of Statistical Sciences, University of Padova, Italy. 4 Division of Biostatistics, University of California at Berkeley, Berkeley, CA 94720, USA. 5 Bioinformatics Division, WEHI, Parkville, VIC 3052, Australia. 6 Department of Biological Sciences, The University of Texas at Dallas, Richardson Texas, USA # Correspondence to: [email protected], [email protected] and [email protected] †These authors contributed equally. Abstract: Retrotransposons mediate gene regulation in multiple developmental and pathological processes. Here, we characterized the transient retrotransposon induction in preimplantation development of eight mammalian species. While species-specific in sequences, induced retrotransposons exhibit a similar preimplantation profile, conferring gene regulatory activities particularly through LTR retrotransposon promoters. We investigated a mouse-specific MT2B2 retrotransposon promoter, which generates an N-terminally truncated, preimplantation-specific Cdk2ap1ΔN isoform to promote cell proliferation. Cdk2ap1ΔN functionally contrasts to the canonical Cdk2ap1, which represses cell proliferation and peaks in mid-gestation stage. -
LINE1 Inhibition (With Nucleoside Reverse Transcriptase Inhibitors)
Cognitive Vitality Reports® are reports written by neuroscientists at the Alzheimer’s Drug Discovery Foundation (ADDF). These scientific reports include analysis of drugs, drugs-in- development, drug targets, supplements, nutraceuticals, food/drink, non-pharmacologic interventions, and risk factors. Neuroscientists evaluate the potential benefit (or harm) for brain health, as well as for age-related health concerns that can affect brain health (e.g., cardiovascular diseases, cancers, diabetes/metabolic syndrome). In addition, these reports include evaluation of safety data, from clinical trials if available, and from preclinical models. LINE1 inhibition (with nucleoside reverse transcriptase inhibitors) Evidence Summary L1 activation may be associated with age-related diseases, and nucleoside reverse transcriptase inhibitors (NRTIs) suppress L1 activation and may be beneficial in age-related diseases. Neuroprotective Benefit: Increased retrotransposition of transposable elements is implicated in neurodegenerative diseases, so NRTIs could theoretically reverse these actions, but only preclinical evidence exists to date. Aging and related health concerns: Preclinical studies suggest that NRTIs may be an effective anti-aging therapy, and clinical studies suggest they may be effective for cancer. Safety: NRTIs are associated with some mild side effects and rare severe, possibly life- threatening, side effects. 1 Availability: Available with Dose: Depends on the Molecular Formula: N/A prescription (as generics) NRTI Molecular weight: N/A Half-life: Depends on the BBB: Possibly penetrant NRTI in animal models Clinical trials: Many for HIV, Observational studies: one for ALS Many What is it? Retrotransposons are stretches of DNA that can replicate and reinsert into other parts of the genome. Their presence in the DNA comes from retroviral infection of germline cells throughout evolutionary history. -
Multiple Non-LTR Retrotransposons in the Genome of Arabidqpsis Thulium
Copyright 0 1996 by the Genetics Society of America Multiple Non-LTR Retrotransposons in the Genome of ArabidqPsis thulium David A. Wright,* Ning Ke," Jan Smalle,t" Brian M. Hauge,t'2Howard M. Goodmant and Daniel F. Voytas* *Department of Zoology and Genetics, Iowa State University, Ames, Iowa 50011 and tDepartment of Genetics, Haward Medical School and Department of Molecular Biology, Massachusetts General Hospital, Boston, Massachusetts 021 14 Manuscript received June 13, 1995 Accepted for publication October 14, 1995 ABSTRACT DNA sequence analysis near the Arabidopsis thaliana AB13 gene revealed the presence of a non-LTR retrotransposon insertion thatwe have designated Tal 1-1.This insertion is 6.2 kb in length and encodes two overlapping reading frames with similarity to non-LTR retrotransposon proteins, including reverse transcriptase. A polymerase chain reaction assaywas developed based on conserved amino acid sequences shared between the Tall-1 reverse transcriptase and those of non-LTR retrotransposons from other species. Seventeen additionalA. thaliana reverse transcriptases were identified that range in nucleotide similarity from 4848% (Ta12-Ta28). Phylogenetic analyses indicated that the A. thaliana sequences are more closely related to each other than to elements from other organisms, consistent with the vertical evolution of these sequences over mostof their evolutionary history. One sequence, Ta17,is located in the mitochondrial genome. The remaining are nuclear andof low copy number among 17 diverse A. thaliana ecotypes tested, suggesting that they are not highly active in transposition. The paucity of retrotransposons and the small genome size of A. thaliana support the hypothesis that most repetitive sequences have been lost from the genome and that mechanisms may exist to prevent amplification of extant element families. -
Sap1 Dependent Replication Fork Barriers Guide Integration of Ltr Retrotransposons in S
SAP1 DEPENDENT REPLICATION FORK BARRIERS GUIDE INTEGRATION OF LTR RETROTRANSPOSONS IN S. POMBE By! JAKE ZACHARY JACOBS A Dissertation submitted to the! Graduate School-New Brunswick! Rutgers, The State University of New Jersey !In partial fulfillment of the requirements! For the degree of !Doctor of Philosophy !Graduate Program in Biochemistry !Written under the direction of Dr. Mikel Zaratiegui, Ph.D.! And approved by _____________________________________________________ _____________________________________________________ _____________________________________________________ _____________________________________________________! New Brunswick, New Jersey! October 2016 ABSTRACT OF THE DISSERTATION SAP1 DEPENDENT REPLICATION FORK BARRIERS GUIDE INTEGRATION OF LTR RETROTRANSPOSONS IN S. POMBE By! JAKE ZACHARY JACOBS Dissertation Director: Dr. Mikel Zaratiegui Long Terminal Repeat retrotransposable elements (LTR-TEs) are a large group of eukaryotic Transposable Elements characterized by flanking repeats in tandem orientation—the LTRs. The LTRs of these elements contain sequences that recruit proteins involved in their expression, replication, silencing, organization, and stability. A successful transposable element must maximize its reproductive amplification without jeopardizing its host, and several characterized LTR-TEs appear to accomplish this through the selection of integration sites away from protein coding sequences. However, despite their high relatedness, a universal mechanism that explains how these parasitic elements avoid -
Genome-Wide Mapping and Characterization of Microsatellites In
www.nature.com/scientificreports OPEN Genome-wide mapping and characterization of microsatellites in the swamp eel genome Received: 14 February 2017 Zhigang Li, Feng Chen, Chunhua Huang, Weixin Zheng, Chunlai Yu, Hanhua Cheng & Accepted: 26 April 2017 Rongjia Zhou Published: xx xx xxxx We described genome-wide screening and characterization of microsatellites in the swamp eel genome. A total of 99,293 microsatellite loci were identified in the genome with an overall density of 179 microsatellites per megabase of genomic sequences. The dinucleotide microsatellites were the most abundant type representing 71% of the total microsatellite loci and the AC-rich motifs were the most recurrent in all repeat types. Microsatellite frequency decreased as numbers of repeat units increased, which was more obvious in long than short microsatellite motifs. Most of microsatellites were located in non-coding regions, whereas only approximately 1% of the microsatellites were detected in coding regions. Trinucleotide repeats were most abundant microsatellites in the coding regions, which represented amino acid repeats in proteins. There was a chromosome-biased distribution of microsatellites in non-coding regions, with the highest density of 203.95/Mb on chromosome 8 and the least on chromosome 7 (164.06/Mb). The most abundant dinucleotides (AC)n was mainly located on chromosome 8. Notably, genomic mapping showed that there was a chromosome-biased association of genomic distributions between microsatellites and transposon elements. Thus, the novel dataset of microsatellites in swamp eel provides a valuable resource for further studies on QTL-based selection breeding, genetic resource conservation and evolutionary genetics. Swamp eel (Monopterus albus) taxonomically belongs to teleosts, the family Synbranchidae of the order Synbranchiformes (Neoteleostei, Teleostei, and Vertebrata). -
Environmental and Epigenetic Regulation of Rider
University of Birmingham Environmental and epigenetic regulation of Rider retrotransposons in tomato Benoit, Matthias; Drost, Hajk-Georg; Catoni, Marco; Gouil, Quentin; Lopez-Gomollon, Sara; Baulcombe, David; Paszkowski, Jerzy DOI: 10.1371/journal.pgen.1008370 License: Creative Commons: Attribution (CC BY) Document Version Publisher's PDF, also known as Version of record Citation for published version (Harvard): Benoit, M, Drost, H-G, Catoni, M, Gouil, Q, Lopez-Gomollon, S, Baulcombe, D & Paszkowski, J 2019, 'Environmental and epigenetic regulation of Rider retrotransposons in tomato', PLoS Genetics, vol. 15, no. 9, e1008370, pp. 1-28. https://doi.org/10.1371/journal.pgen.1008370 Link to publication on Research at Birmingham portal Publisher Rights Statement: Benoit, M, Drost, H-G, Catoni, M, Gouil, Q, Lopez-Gomollon, S, Baulcombe, D & Paszkowski, J 2019, 'Environmental and epigenetic regulation of Rider retrotransposons in tomato', PLoS Genetics, vol. 15, no. 9, e1008370, pp. 1-28. https://doi.org/10.1371/journal.pgen.1008370 © 2019 Benoit et al. General rights Unless a licence is specified above, all rights (including copyright and moral rights) in this document are retained by the authors and/or the copyright holders. The express permission of the copyright holder must be obtained for any use of this material other than for purposes permitted by law. •Users may freely distribute the URL that is used to identify this publication. •Users may download and/or print one copy of the publication from the University of Birmingham research portal for the purpose of private study or non-commercial research. •User may use extracts from the document in line with the concept of ‘fair dealing’ under the Copyright, Designs and Patents Act 1988 (?) •Users may not further distribute the material nor use it for the purposes of commercial gain. -
Solanum Section Lycopersicon: Solanaceae)
Biological Journal of the Linnean Society, 2016, 117, 96–105. With 4 figures. Using genomic repeats for phylogenomics: a case study in wild tomatoes (Solanum section Lycopersicon: Solanaceae) 1,2 2,3 € 4 5 STEVEN DODSWORTH *, MARK W. CHASE , TIINA SARKINEN , SANDRA KNAPP and ANDREW R. LEITCH1 1School of Biological and Chemical Sciences, Queen Mary University of London, Mile End Road, London, E1 4NS, UK 2Royal Botanic Gardens, Kew, Richmond, Surrey, TW9 3DS, UK 3School of Plant Biology, The University of Western Australia, Crawley, WA, 6009, Australia 4Royal Botanic Garden, Edinburgh, 20A Inverleith Row, Edinburgh, EH3 5LR, UK 5Department of Life Sciences, Natural History Museum, Cromwell Road, London, SW7 5BD, UK Received 17 February 2015; revised 7 May 2015; accepted for publication 21 May 2015 High-throughput sequencing data have transformed molecular phylogenetics and a plethora of phylogenomic approaches are now readily available. Shotgun sequencing at low genome coverage is a common approach for isolating high-copy DNA, such as the plastid or mitochondrial genomes, and ribosomal DNA. These sequence data, however, are also rich in repetitive elements that are often discarded. Such data include a variety of repeats present throughout the nuclear genome in high copy number. It has recently been shown that the abundance of repetitive elements has phylogenetic signal and can be used as a continuous character to infer tree topologies. In the present study, we evaluate repetitive DNA data in tomatoes (Solanum section Lycopersicon)to explore how they perform at the inter- and intraspecific levels, utilizing the available data from the 100 Tomato Genome Sequencing Consortium. -
Downloads/Repeatmaskedgenomes
Kojima Mobile DNA (2018) 9:2 DOI 10.1186/s13100-017-0107-y REVIEW Open Access Human transposable elements in Repbase: genomic footprints from fish to humans Kenji K. Kojima1,2 Abstract Repbase is a comprehensive database of eukaryotic transposable elements (TEs) and repeat sequences, containing over 1300 human repeat sequences. Recent analyses of these repeat sequences have accumulated evidences for their contribution to human evolution through becoming functional elements, such as protein-coding regions or binding sites of transcriptional regulators. However, resolving the origins of repeat sequences is a challenge, due to their age, divergence, and degradation. Ancient repeats have been continuously classified as TEs by finding similar TEs from other organisms. Here, the most comprehensive picture of human repeat sequences is presented. The human genome contains traces of 10 clades (L1, CR1, L2, Crack, RTE, RTEX, R4, Vingi, Tx1 and Penelope) of non-long terminal repeat (non-LTR) retrotransposons (long interspersed elements, LINEs), 3 types (SINE1/7SL, SINE2/tRNA, and SINE3/5S) of short interspersed elements (SINEs), 1 composite retrotransposon (SVA) family, 5 classes (ERV1, ERV2, ERV3, Gypsy and DIRS) of LTR retrotransposons, and 12 superfamilies (Crypton, Ginger1, Harbinger, hAT, Helitron, Kolobok, Mariner, Merlin, MuDR, P, piggyBac and Transib) of DNA transposons. These TE footprints demonstrate an evolutionary continuum of the human genome. Keywords: Human repeat, Transposable elements, Repbase, Non-LTR retrotransposons, LTR retrotransposons, DNA transposons, SINE, Crypton, MER, UCON Background contrast, MER4 was revealed to be comprised of LTRs of Repbase and conserved noncoding elements endogenous retroviruses (ERVs) [1]. Right now, Repbase Repbase is now one of the most comprehensive data- keeps MER1 to MER136, some of which are further bases of eukaryotic transposable elements and repeats divided into several subfamilies. -
Drosophila: Retrotransposons Making up Telomeres
Review Drosophila: Retrotransposons Making up Telomeres Elena Casacuberta Institute of Evolutionary Biology, IBE, CSIC—Pompeu Fabra University, Barcelona Spain, Passeig de la Barceloneta 37‐49, 08003 Barcelona, Spain; [email protected]‐csic.es; Tel.: +34‐932309637; Fax: +34‐932309555 Guest Editors: Paul M. Liebermann and Benedikt B. Kaufer Received: 9 June 2017; Accepted: 17 July 2017; Published: 19 July 2017 Abstract: Drosophila and extant species are the best‐studied telomerase exception. In this organism, telomere elongation is coupled with targeted retrotransposition of Healing Transposon (HeT‐A) and Telomere Associated Retrotransposon (TART) with sporadic additions of Telomere Associated and HeT‐A Related (TAHRE), all three specialized non‐Long Terminal Repeat (non‐LTR) retrotransposons. These three very special retroelements transpose in head to tail arrays, always in the same orientation at the end of the chromosomes but never in interior locations. Apparently, retrotransposon and telomerase telomeres might seem very different, but a detailed view of their mechanisms reveals similarities explaining how the loss of telomerase in a Drosophila ancestor could successfully have been replaced by the telomere retrotransposons. In this review, we will discover that although HeT‐A, TART, and TAHRE are still the only examples to date where their targeted transposition is perfectly tamed into the telomere biology of Drosophila, there are other examples of retrotransposons that manage to successfully integrate inside and at the end of telomeres. Because the aim of this special issue is viral integration at telomeres, understanding the base of the telomerase exceptions will help to obtain clues on similar strategies that mobile elements and viruses could have acquired in order to ensure their survival in the host genome. -
The Sequenced Angiosperm Genomes and Genome Databases
REVIEW published: 13 April 2018 doi: 10.3389/fpls.2018.00418 The Sequenced Angiosperm Genomes and Genome Databases Fei Chen 1†, Wei Dong 1†, Jiawei Zhang 1, Xinyue Guo 1, Junhao Chen 2, Zhengjia Wang 2, Zhenguo Lin 3, Haibao Tang 1 and Liangsheng Zhang 1* 1 State Key Laboratory of Ecological Pest Control for Fujian and Taiwan Crops, College of Life Sciences, Fujian Provincial Key Laboratory of Haixia Applied Plant Systems Biology, Ministry of Education Key Laboratory of Genetics, Breeding and Multiple Utilization of Corps, Fujian Agriculture and Forestry University, Fuzhou, China, 2 State Key Laboratory of Subtropical Silviculture, School of Forestry and Biotechnology, Zhejiang Agriculture and Forestry University, Hangzhou, China, 3 Department of Biology, Saint Louis University, St. Louis, MO, United States Angiosperms, the flowering plants, provide the essential resources for human life, such as food, energy, oxygen, and materials. They also promoted the evolution of human, animals, and the planet earth. Despite the numerous advances in genome reports or sequencing technologies, no review covers all the released angiosperm genomes and the genome databases for data sharing. Based on the rapid advances and innovations in the database reconstruction in the last few years, here we provide a comprehensive Edited by: review for three major types of angiosperm genome databases, including databases Santosh Kumar Upadhyay, Panjab University, India for a single species, for a specific angiosperm clade, and for multiple angiosperm Reviewed by: species. The scope, tools, and data of each type of databases and their features Sumit Kumar Bag, are concisely discussed. The genome databases for a single species or a clade of National Botanical Research Institute species are especially popular for specific group of researchers, while a timely-updated (CSIR), India Xiyin Wang, comprehensive database is more powerful for address of major scientific mysteries at North China University of Science and the genome scale.