An Actin-Related Protein That Is Most Highly Expressed in Drosophila
Total Page:16
File Type:pdf, Size:1020Kb
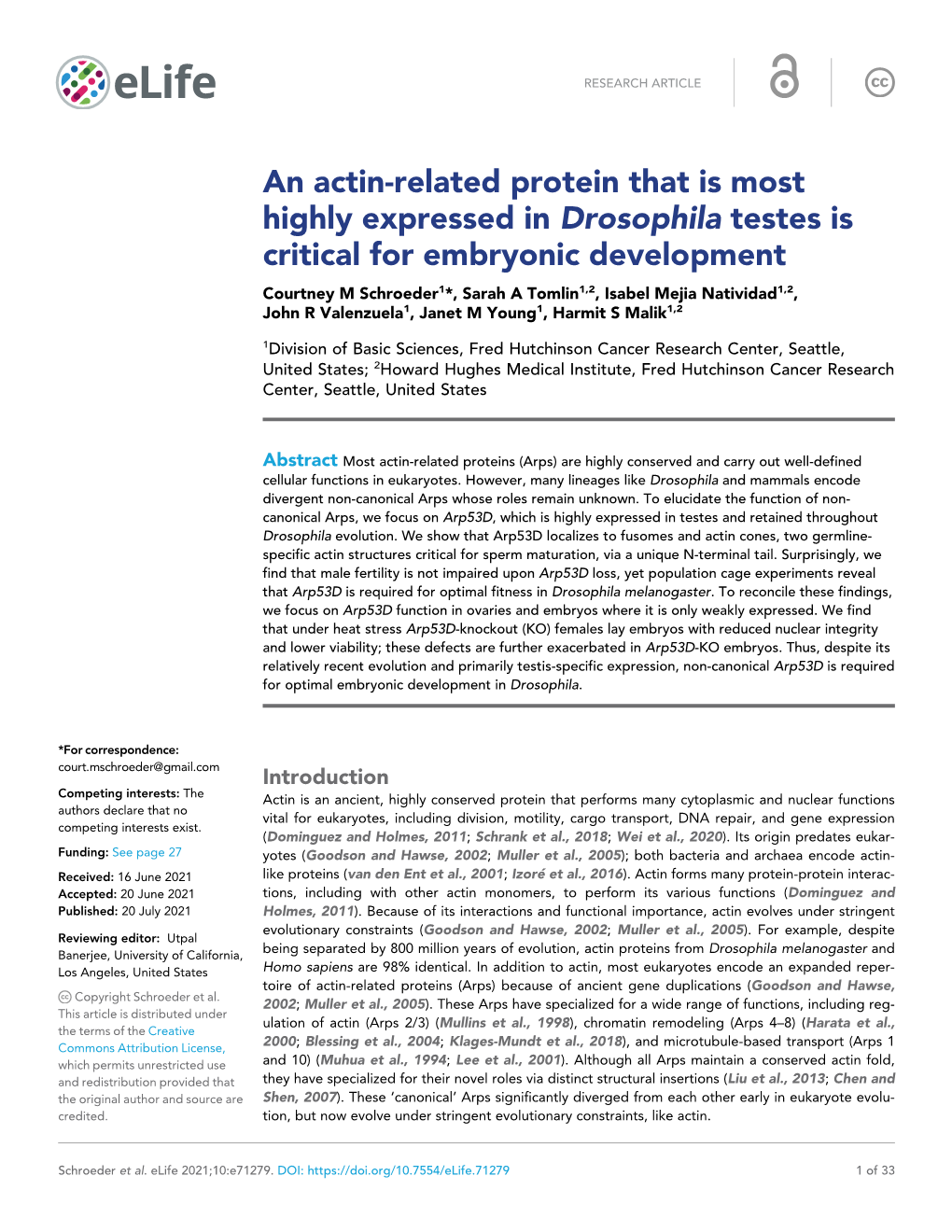
Load more
Recommended publications
-
Ovarian Gene Expression in the Absence of FIGLA, an Oocyte
BMC Developmental Biology BioMed Central Research article Open Access Ovarian gene expression in the absence of FIGLA, an oocyte-specific transcription factor Saurabh Joshi*1, Holly Davies1, Lauren Porter Sims2, Shawn E Levy2 and Jurrien Dean1 Address: 1Laboratory of Cellular and Developmental Biology, NIDDK, National Institutes of Health, Bethesda, MD 20892, USA and 2Department of Biomedical Informatics, Vanderbilt University Medical Center, Nashville, TN 37232, USA Email: Saurabh Joshi* - [email protected]; Holly Davies - [email protected]; Lauren Porter Sims - [email protected]; Shawn E Levy - [email protected]; Jurrien Dean - [email protected] * Corresponding author Published: 13 June 2007 Received: 11 December 2006 Accepted: 13 June 2007 BMC Developmental Biology 2007, 7:67 doi:10.1186/1471-213X-7-67 This article is available from: http://www.biomedcentral.com/1471-213X/7/67 © 2007 Joshi et al; licensee BioMed Central Ltd. This is an Open Access article distributed under the terms of the Creative Commons Attribution License (http://creativecommons.org/licenses/by/2.0), which permits unrestricted use, distribution, and reproduction in any medium, provided the original work is properly cited. Abstract Background: Ovarian folliculogenesis in mammals is a complex process involving interactions between germ and somatic cells. Carefully orchestrated expression of transcription factors, cell adhesion molecules and growth factors are required for success. We have identified a germ-cell specific, basic helix-loop-helix transcription factor, FIGLA (Factor In the GermLine, Alpha) and demonstrated its involvement in two independent developmental processes: formation of the primordial follicle and coordinate expression of zona pellucida genes. Results: Taking advantage of Figla null mouse lines, we have used a combined approach of microarray and Serial Analysis of Gene Expression (SAGE) to identify potential downstream target genes. -
Whole Exome Sequencing in Families at High Risk for Hodgkin Lymphoma: Identification of a Predisposing Mutation in the KDR Gene
Hodgkin Lymphoma SUPPLEMENTARY APPENDIX Whole exome sequencing in families at high risk for Hodgkin lymphoma: identification of a predisposing mutation in the KDR gene Melissa Rotunno, 1 Mary L. McMaster, 1 Joseph Boland, 2 Sara Bass, 2 Xijun Zhang, 2 Laurie Burdett, 2 Belynda Hicks, 2 Sarangan Ravichandran, 3 Brian T. Luke, 3 Meredith Yeager, 2 Laura Fontaine, 4 Paula L. Hyland, 1 Alisa M. Goldstein, 1 NCI DCEG Cancer Sequencing Working Group, NCI DCEG Cancer Genomics Research Laboratory, Stephen J. Chanock, 5 Neil E. Caporaso, 1 Margaret A. Tucker, 6 and Lynn R. Goldin 1 1Genetic Epidemiology Branch, Division of Cancer Epidemiology and Genetics, National Cancer Institute, NIH, Bethesda, MD; 2Cancer Genomics Research Laboratory, Division of Cancer Epidemiology and Genetics, National Cancer Institute, NIH, Bethesda, MD; 3Ad - vanced Biomedical Computing Center, Leidos Biomedical Research Inc.; Frederick National Laboratory for Cancer Research, Frederick, MD; 4Westat, Inc., Rockville MD; 5Division of Cancer Epidemiology and Genetics, National Cancer Institute, NIH, Bethesda, MD; and 6Human Genetics Program, Division of Cancer Epidemiology and Genetics, National Cancer Institute, NIH, Bethesda, MD, USA ©2016 Ferrata Storti Foundation. This is an open-access paper. doi:10.3324/haematol.2015.135475 Received: August 19, 2015. Accepted: January 7, 2016. Pre-published: June 13, 2016. Correspondence: [email protected] Supplemental Author Information: NCI DCEG Cancer Sequencing Working Group: Mark H. Greene, Allan Hildesheim, Nan Hu, Maria Theresa Landi, Jennifer Loud, Phuong Mai, Lisa Mirabello, Lindsay Morton, Dilys Parry, Anand Pathak, Douglas R. Stewart, Philip R. Taylor, Geoffrey S. Tobias, Xiaohong R. Yang, Guoqin Yu NCI DCEG Cancer Genomics Research Laboratory: Salma Chowdhury, Michael Cullen, Casey Dagnall, Herbert Higson, Amy A. -
Goat Anti-Actin-Like 7B Antibody Peptide-Affinity Purified Goat Antibody Catalog # Af1021b
10320 Camino Santa Fe, Suite G San Diego, CA 92121 Tel: 858.875.1900 Fax: 858.622.0609 Goat Anti-Actin-like 7B Antibody Peptide-affinity purified goat antibody Catalog # AF1021b Specification Goat Anti-Actin-like 7B Antibody - Product Information Application WB Primary Accession Q9Y614 Other Accession NP_006677, 10880 Reactivity Human Host Goat Clonality Polyclonal Concentration 100ug/200ul Isotype IgG Calculated MW 45234 Goat Anti-Actin-like 7B Antibody - Additional AF1021b (0.03 µg/ml) staining of human Information testis lysate (35 µg protein in RIPA buffer). Primary incubation was 1 hour. Detected by Gene ID 10880 chemiluminescence. Other Names Actin-like protein 7B, Actin-like-7-beta, Goat Anti-Actin-like 7B Antibody - ACTL7B Background Format The protein encoded by this gene is a member 0.5 mg IgG/ml in Tris saline (20mM Tris of a family of actin-related proteins (ARPs) pH7.3, 150mM NaCl), 0.02% sodium azide, which share significant amino acid sequence with 0.5% bovine serum albumin identity to conventional actins. Both actins and ARPs have an actin fold, which is an Storage ATP-binding cleft, as a common feature. The Maintain refrigerated at 2-8°C for up to 6 ARPs are involved in diverse cellular processes, months. For long term storage store at including vesicular transport, spindle -20°C in small aliquots to prevent orientation, nuclear migration and chromatin freeze-thaw cycles. remodeling. This gene (ACTL7B), and related gene, ACTL7A, are intronless, and are located Precautions Goat Anti-Actin-like 7B Antibody is for approximately 4 kb apart in a head-to-head research use only and not for use in orientation within the familial dysautonomia diagnostic or therapeutic procedures. -
Genome-Wide Association Studies of Smooth Pursuit and Antisaccade Eye Movements in Psychotic Disorders: findings from the B-SNIP Study
OPEN Citation: Transl Psychiatry (2017) 7, e1249; doi:10.1038/tp.2017.210 www.nature.com/tp ORIGINAL ARTICLE Genome-wide association studies of smooth pursuit and antisaccade eye movements in psychotic disorders: findings from the B-SNIP study R Lencer1, LJ Mills2, N Alliey-Rodriguez3, R Shafee4,5,AMLee6, JL Reilly7, A Sprenger8, JE McDowell9, SA McCarroll4, MS Keshavan10, GD Pearlson11,12, CA Tamminga13, BA Clementz9, ES Gershon3, JA Sweeney13,14 and JR Bishop6,15 Eye movement deviations, particularly deficits of initial sensorimotor processing and sustained pursuit maintenance, and antisaccade inhibition errors, are established intermediate phenotypes for psychotic disorders. We here studied eye movement measures of 849 participants from the Bipolar-Schizophrenia Network on Intermediate Phenotypes (B-SNIP) study (schizophrenia N = 230, schizoaffective disorder N = 155, psychotic bipolar disorder N = 206 and healthy controls N = 258) as quantitative phenotypes in relation to genetic data, while controlling for genetically derived ancestry measures, age and sex. A mixed-modeling genome-wide association studies approach was used including ~ 4.4 million genotypes (PsychChip and 1000 Genomes imputation). Across participants, sensorimotor processing at pursuit initiation was significantly associated with a single nucleotide polymorphism in IPO8 (12p11.21, P =8×10− 11), whereas suggestive associations with sustained pursuit maintenance were identified with SNPs in SH3GL2 (9p22.2, P =3×10− 8). In participants of predominantly African ancestry, sensorimotor processing was also significantly associated with SNPs in PCDH12 (5q31.3, P = 1.6 × 10 − 10), and suggestive associations were observed with NRSN1 (6p22.3, P = 5.4 × 10 −8) and LMO7 (13q22.2, P = 7.3x10−8), whereas antisaccade error rate was significantly associated with a non-coding region at chromosome 7 (P = 6.5 × 10− 9). -
Methylation of Leukocyte DNA and Ovarian Cancer
Fridley et al. BMC Medical Genomics 2014, 7:21 http://www.biomedcentral.com/1755-8794/7/21 RESEARCH ARTICLE Open Access Methylation of leukocyte DNA and ovarian cancer: relationships with disease status and outcome Brooke L Fridley1*, Sebastian M Armasu2, Mine S Cicek2, Melissa C Larson2, Chen Wang2, Stacey J Winham2, Kimberly R Kalli3, Devin C Koestler1,4, David N Rider2, Viji Shridhar5, Janet E Olson2, Julie M Cunningham5 and Ellen L Goode2 Abstract Background: Genome-wide interrogation of DNA methylation (DNAm) in blood-derived leukocytes has become feasible with the advent of CpG genotyping arrays. In epithelial ovarian cancer (EOC), one report found substantial DNAm differences between cases and controls; however, many of these disease-associated CpGs were attributed to differences in white blood cell type distributions. Methods: We examined blood-based DNAm in 336 EOC cases and 398 controls; we included only high-quality CpG loci that did not show evidence of association with white blood cell type distributions to evaluate association with case status and overall survival. Results: Of 13,816 CpGs, no significant associations were observed with survival, although eight CpGs associated with survival at p < 10−3, including methylation within a CpG island located in the promoter region of GABRE (p = 5.38 x 10−5, HR = 0.95). In contrast, 53 CpG methylation sites were significantly associated with EOC risk (p <5 x10−6). The top association was observed for the methylation probe cg04834572 located approximately 315 kb upstream of DUSP13 (p = 1.6 x10−14). Other disease-associated CpGs included those near or within HHIP (cg14580567; p =5.6x10−11), HDAC3 (cg10414058; p = 6.3x10−12), and SCR (cg05498681; p = 4.8x10−7). -
A Rapidly Evolving Actin Mediates Fertility and Developmental Tradeoffs in Drosophila
bioRxiv preprint doi: https://doi.org/10.1101/2020.09.28.317503; this version posted September 28, 2020. The copyright holder for this preprint (which was not certified by peer review) is the author/funder, who has granted bioRxiv a license to display the preprint in perpetuity. It is made available under aCC-BY-NC-ND 4.0 International license. 1 A rapidly evolving actin mediates fertility and developmental tradeoffs in Drosophila Courtney M. Schroeder1,*, Sarah A. Tomlin1,2, John R. Valenzuela1, and Harmit S. Malik1,2,* 1Division of Basic Sciences & 2Howard HuGhes Medical Institute, Fred Hutchinson Cancer Research Center, Seattle, WA RunninG Title: Actin paraloG Arp53D plays opposinG roles in fertility and development *Correspondence should be addressed to: Courtney Schroeder, 1100 Fairview Avenue N. A2-025, Seattle, WA 98109, [email protected]; Harmit S. Malik, 1100 Fairview Avenue N. A2-205, Seattle, WA 98109, [email protected] bioRxiv preprint doi: https://doi.org/10.1101/2020.09.28.317503; this version posted September 28, 2020. The copyright holder for this preprint (which was not certified by peer review) is the author/funder, who has granted bioRxiv a license to display the preprint in perpetuity. It is made available under aCC-BY-NC-ND 4.0 International license. 2 Abstract Most actin-related proteins (Arps) are highly conserved in eukaryotes, where they carry out well-defined cellular functions. Drosophila and mammals also encode divergent non- canonical Arps in their male-germline whose roles remain unknown. Here, we show that Arp53D, a rapidly-evolving Drosophila Arp, localizes to fusomes and actin cones, two male germline-specific actin structures critical for sperm maturation, via its non- canonical N-terminal tail. -
Supercomputing Pipeline for the Ark (SPARK) Thesis
Supercomputing Pipeline for The Ark (SPARK) Thilina Sunimal Ranaweera Submitted in total fulfilment of the requirements of the degree of Doctor of Philosophy Melbourne School of Population and Global Health The University of Melbourne August 2018 Produced on archival quality paper. Copyright c 2018 Thilina Sunimal Ranaweera All rights reserved. No part of the publication may be reproduced in any form by print, photoprint, microfilm or any other means without written permission from the author. Abstract Data is the primary asset of biomedical researchers and the engine for both dis- covery and translation. The challenge is to facilitate the discovery of new medical knowledge, supported by advanced computing technologies based on heterogeneous medical datasets. At the beginning of the 21st century, the much-anticipated Hu- man Genome Project was completed, thus commencing an era of high-dimensional genomic datasets. Additionally, the internet era has introduced advanced and more user-friendly computing technologies for data storage, processing, and analysis. The biomedical informatics research field has emerged to fill the void between medical research and computing technologies. The Ark is an open-source web-based medical research data management plat- form which currently supports multiple medical research studies. The Ark’s soft- ware architecture is modular, capable of managing multiple studies and sub-studies in a single database, including laboratory information and questionnaire data. The Ark’s role-based security infrastructure provides a secure environment for biomed- ical datasets. Prior to the work presented in this thesis, The Ark did not have the capability to effectively manage or analyse genomic data, nor model pedigree structures. -
The Human Gene Connectome As a Map of Short Cuts for Morbid Allele Discovery
The human gene connectome as a map of short cuts for morbid allele discovery Yuval Itana,1, Shen-Ying Zhanga,b, Guillaume Vogta,b, Avinash Abhyankara, Melina Hermana, Patrick Nitschkec, Dror Friedd, Lluis Quintana-Murcie, Laurent Abela,b, and Jean-Laurent Casanovaa,b,f aSt. Giles Laboratory of Human Genetics of Infectious Diseases, Rockefeller Branch, The Rockefeller University, New York, NY 10065; bLaboratory of Human Genetics of Infectious Diseases, Necker Branch, Paris Descartes University, Institut National de la Santé et de la Recherche Médicale U980, Necker Medical School, 75015 Paris, France; cPlateforme Bioinformatique, Université Paris Descartes, 75116 Paris, France; dDepartment of Computer Science, Ben-Gurion University of the Negev, Beer-Sheva 84105, Israel; eUnit of Human Evolutionary Genetics, Centre National de la Recherche Scientifique, Unité de Recherche Associée 3012, Institut Pasteur, F-75015 Paris, France; and fPediatric Immunology-Hematology Unit, Necker Hospital for Sick Children, 75015 Paris, France Edited* by Bruce Beutler, University of Texas Southwestern Medical Center, Dallas, TX, and approved February 15, 2013 (received for review October 19, 2012) High-throughput genomic data reveal thousands of gene variants to detect a single mutated gene, with the other polymorphic genes per patient, and it is often difficult to determine which of these being of less interest. This goes some way to explaining why, variants underlies disease in a given individual. However, at the despite the abundance of NGS data, the discovery of disease- population level, there may be some degree of phenotypic homo- causing alleles from such data remains somewhat limited. geneity, with alterations of specific physiological pathways under- We developed the human gene connectome (HGC) to over- come this problem. -
Epigenetic Mechanisms Are Involved in the Oncogenic Properties of ZNF518B in Colorectal Cancer
Epigenetic mechanisms are involved in the oncogenic properties of ZNF518B in colorectal cancer Francisco Gimeno-Valiente, Ángela L. Riffo-Campos, Luis Torres, Noelia Tarazona, Valentina Gambardella, Andrés Cervantes, Gerardo López-Rodas, Luis Franco and Josefa Castillo SUPPLEMENTARY METHODS 1. Selection of genomic sequences for ChIP analysis To select the sequences for ChIP analysis in the five putative target genes, namely, PADI3, ZDHHC2, RGS4, EFNA5 and KAT2B, the genomic region corresponding to the gene was downloaded from Ensembl. Then, zoom was applied to see in detail the promoter, enhancers and regulatory sequences. The details for HCT116 cells were then recovered and the target sequences for factor binding examined. Obviously, there are not data for ZNF518B, but special attention was paid to the target sequences of other zinc-finger containing factors. Finally, the regions that may putatively bind ZNF518B were selected and primers defining amplicons spanning such sequences were searched out. Supplementary Figure S3 gives the location of the amplicons used in each gene. 2. Obtaining the raw data and generating the BAM files for in silico analysis of the effects of EHMT2 and EZH2 silencing The data of siEZH2 (SRR6384524), siG9a (SRR6384526) and siNon-target (SRR6384521) in HCT116 cell line, were downloaded from SRA (Bioproject PRJNA422822, https://www.ncbi. nlm.nih.gov/bioproject/), using SRA-tolkit (https://ncbi.github.io/sra-tools/). All data correspond to RNAseq single end. doBasics = TRUE doAll = FALSE $ fastq-dump -I --split-files SRR6384524 Data quality was checked using the software fastqc (https://www.bioinformatics.babraham. ac.uk /projects/fastqc/). The first low quality removing nucleotides were removed using FASTX- Toolkit (http://hannonlab.cshl.edu/fastxtoolkit/). -
The Human Gene Connectome As a Map of Short Cuts for Morbid Allele Discovery
The human gene connectome as a map of short cuts for morbid allele discovery Yuval Itana,1, Shen-Ying Zhanga,b, Guillaume Vogta,b, Avinash Abhyankara, Melina Hermana, Patrick Nitschkec, Dror Friedd, Lluis Quintana-Murcie, Laurent Abela,b, and Jean-Laurent Casanovaa,b,f aSt. Giles Laboratory of Human Genetics of Infectious Diseases, Rockefeller Branch, The Rockefeller University, New York, NY 10065; bLaboratory of Human Genetics of Infectious Diseases, Necker Branch, Paris Descartes University, Institut National de la Santé et de la Recherche Médicale U980, Necker Medical School, 75015 Paris, France; cPlateforme Bioinformatique, Université Paris Descartes, 75116 Paris, France; dDepartment of Computer Science, Ben-Gurion University of the Negev, Beer-Sheva 84105, Israel; eUnit of Human Evolutionary Genetics, Centre National de la Recherche Scientifique, Unité de Recherche Associée 3012, Institut Pasteur, F-75015 Paris, France; and fPediatric Immunology-Hematology Unit, Necker Hospital for Sick Children, 75015 Paris, France Edited* by Bruce Beutler, University of Texas Southwestern Medical Center, Dallas, TX, and approved February 15, 2013 (received for review October 19, 2012) High-throughput genomic data reveal thousands of gene variants to detect a single mutated gene, with the other polymorphic genes per patient, and it is often difficult to determine which of these being of less interest. This goes some way to explaining why, variants underlies disease in a given individual. However, at the despite the abundance of NGS data, the discovery of disease- population level, there may be some degree of phenotypic homo- causing alleles from such data remains somewhat limited. geneity, with alterations of specific physiological pathways under- We developed the human gene connectome (HGC) to over- come this problem. -
Isolation and Identification of Proteins from Swine Sperm Chromatin and Nuclear Matrix
DOI: 10.21451/1984-3143-AR816 Anim. Reprod., v.14, n.2, p.418-428, Apr./Jun. 2017 Isolation and identification of proteins from swine sperm chromatin and nuclear matrix Guilherme Arantes Mendonça1,3, Romualdo Morandi Filho2, Elisson Terêncio Souza2, Thais Schwarz Gaggini1, Marina Cruvinel Assunção Silva-Mendonça1, Robson Carlos Antunes1, Marcelo Emílio Beletti1,2 1Post-graduation Program in Veterinary Science, Federal University of Uberlandia, Uberlandia, MG, Brazil. 2Post-graduation Program in Cellular and Molecular Biology, Federal University of Uberlandia, Uberlandia, MG, Brazil. Abstract (Yamauchi et al., 2011). According to the same authors, these active sperm chromatin sites in protamine toroids The aim of this study was to perform a may contain important epigenetic information for the proteomic analysis to isolate and identify proteins from developing embryo. the swine sperm nuclear matrix to contribute to a The isolated use of genomic and transcriptomic database of swine sperm nuclear proteins. We used pre- information may be insufficient to fully understand a chilled diluted semen from seven boars (19 to 24 week- complex organism because proteomics and old) from the commercial line Landrace x Large White transcriptomics can be discordant and DNA-RNA x Pietran. The semen was processed to separate the relationships cannot be fully correlated. Thus, sperm heads and extract the chromatin and nuclear measurements of other metabolic levels should also be matrix for protein quantification and analysis by mass obtained, such as the study of proteins (Wright et al., spectrometry, by LTQ Orbitrap ELITE mass 2012). According to these same authors, large-scale spectrometer (Thermo-Finnigan) coupled to a nanoflow protein research in organisms (i.e., the proteome-protein chromatography system (LC-MS/MS). -
Content Based Search in Gene Expression Databases and a Meta-Analysis of Host Responses to Infection
Content Based Search in Gene Expression Databases and a Meta-analysis of Host Responses to Infection A Thesis Submitted to the Faculty of Drexel University by Francis X. Bell in partial fulfillment of the requirements for the degree of Doctor of Philosophy November 2015 c Copyright 2015 Francis X. Bell. All Rights Reserved. ii Acknowledgments I would like to acknowledge and thank my advisor, Dr. Ahmet Sacan. Without his advice, support, and patience I would not have been able to accomplish all that I have. I would also like to thank my committee members and the Biomed Faculty that have guided me. I would like to give a special thanks for the members of the bioinformatics lab, in particular the members of the Sacan lab: Rehman Qureshi, Daisy Heng Yang, April Chunyu Zhao, and Yiqian Zhou. Thank you for creating a pleasant and friendly environment in the lab. I give the members of my family my sincerest gratitude for all that they have done for me. I cannot begin to repay my parents for their sacrifices. I am eternally grateful for everything they have done. The support of my sisters and their encouragement gave me the strength to persevere to the end. iii Table of Contents LIST OF TABLES.......................................................................... vii LIST OF FIGURES ........................................................................ xiv ABSTRACT ................................................................................ xvii 1. A BRIEF INTRODUCTION TO GENE EXPRESSION............................. 1 1.1 Central Dogma of Molecular Biology........................................... 1 1.1.1 Basic Transfers .......................................................... 1 1.1.2 Uncommon Transfers ................................................... 3 1.2 Gene Expression ................................................................. 4 1.2.1 Estimating Gene Expression ............................................ 4 1.2.2 DNA Microarrays ......................................................