Behavior of Low-Mass X-Ray Binaries and Their Formation in Globular Clusters
Total Page:16
File Type:pdf, Size:1020Kb
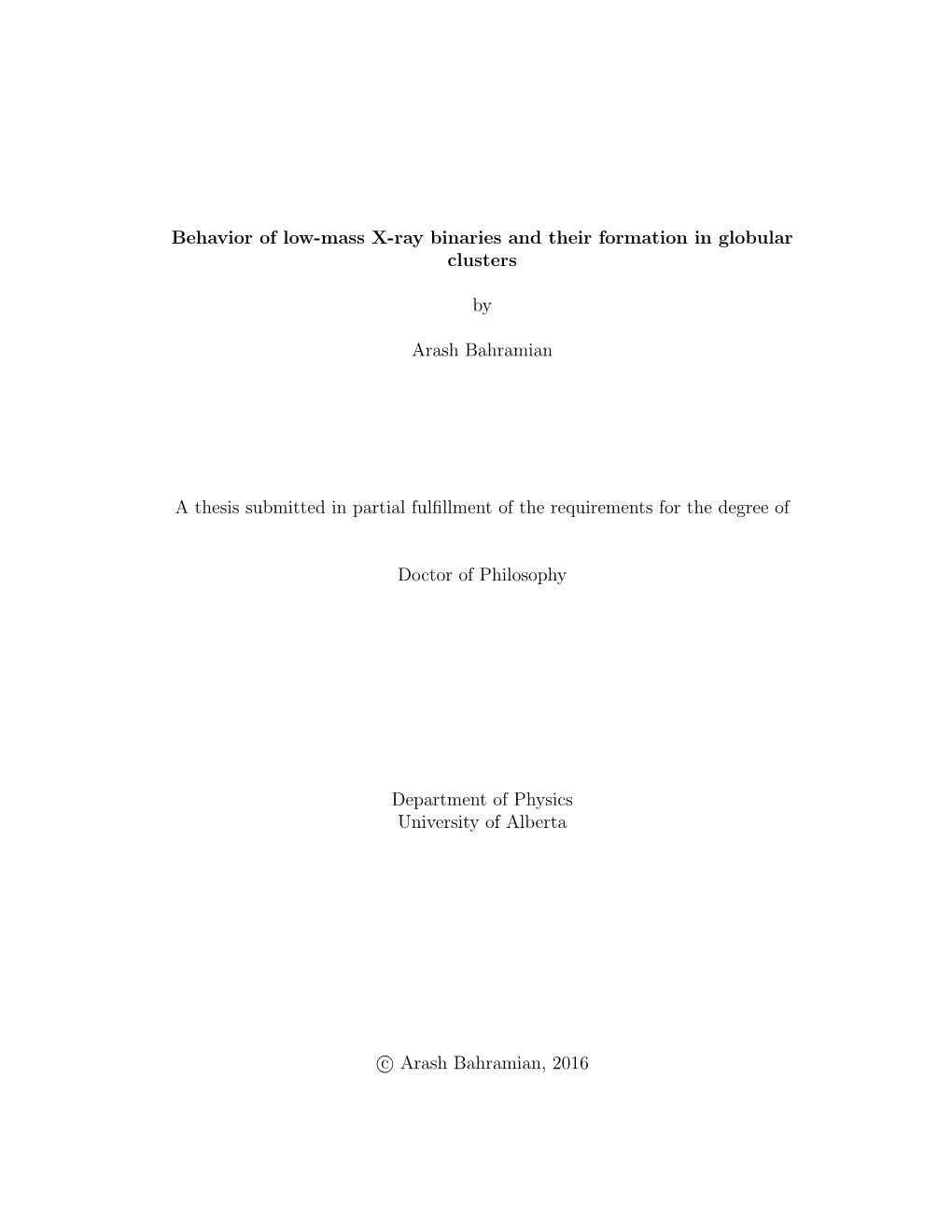
Load more
Recommended publications
-
Tidal Disruption of Globular Clusters in Dwarf Galaxies with Triaxial Dark Matter Haloes.” by J
“Tidal disruption of globular clusters in dwarf galaxies with triaxial dark matter haloes.” by J. Peñarrubia, M.J. Walker, and G. Gilmore University of Cambridge and University of Victoria MNRAS accepted Journal Club 5/15/09 Daniel Grin 1/19 Wednesday, September 2, 2009 1 Punchline 2/19 • Globular clusters (GCs) around dwarf spheroidal (dSph) galaxies may survive tidal encounters • Stellar substructure (morphology and kinematics) in dSph galaxies may be explained by past disruptions of GCs • Simulation techniques grossly over-simplify the problem, but useful first step Wednesday, September 2, 2009 2 Outline 3/19 • Motivation: subtructure in dSphs and existence of GCs near them. • Properties of systems modeled • Simulation techniques/caveats • Results Wednesday, September 2, 2009 3 Motivation: Substructure in dSph galaxies 4/19 • Milky Way (MW) dSph galaxies are DM dominated-- : • Ideal testbed for CDM scenario • Standing dispute about presence of cores (triaxiality? projection effect? see work by J. Simon) • dSph galaxies have substructure, contrary to expectation that it should be erased in a few crossing times (~100 Myr): • Morphology: Kinematically cold core in Sextans, kinematically distinct shell in Fornax, asymmetries across major/minor axes in Fornax (claims of butterfly shapes are sketchy) • Ages: 2 Gyr-old stellar populations in Fornax shell • Can prolong life of stellar substructure with cored density profile, challenge to CDM? • Can save CDM with formation of substructure that is not in situ • Blue stragglers in Sextans are mass segregated, not enough time for this in Sextans, do it elsewhere (like a merging G.C.) and disrupt? Wednesday, September 2, 2009 4 Motivation: Globular clusters in dSph 5/19 • Fornax (5) and Sagitarrius (4) contain GCs near : Name Angular sep. -
Spatial Distribution of Galactic Globular Clusters: Distance Uncertainties and Dynamical Effects
Juliana Crestani Ribeiro de Souza Spatial Distribution of Galactic Globular Clusters: Distance Uncertainties and Dynamical Effects Porto Alegre 2017 Juliana Crestani Ribeiro de Souza Spatial Distribution of Galactic Globular Clusters: Distance Uncertainties and Dynamical Effects Dissertação elaborada sob orientação do Prof. Dr. Eduardo Luis Damiani Bica, co- orientação do Prof. Dr. Charles José Bon- ato e apresentada ao Instituto de Física da Universidade Federal do Rio Grande do Sul em preenchimento do requisito par- cial para obtenção do título de Mestre em Física. Porto Alegre 2017 Acknowledgements To my parents, who supported me and made this possible, in a time and place where being in a university was just a distant dream. To my dearest friends Elisabeth, Robert, Augusto, and Natália - who so many times helped me go from "I give up" to "I’ll try once more". To my cats Kira, Fen, and Demi - who lazily join me in bed at the end of the day, and make everything worthwhile. "But, first of all, it will be necessary to explain what is our idea of a cluster of stars, and by what means we have obtained it. For an instance, I shall take the phenomenon which presents itself in many clusters: It is that of a number of lucid spots, of equal lustre, scattered over a circular space, in such a manner as to appear gradually more compressed towards the middle; and which compression, in the clusters to which I allude, is generally carried so far, as, by imperceptible degrees, to end in a luminous center, of a resolvable blaze of light." William Herschel, 1789 Abstract We provide a sample of 170 Galactic Globular Clusters (GCs) and analyse its spatial distribution properties. -
The NTT Provides the Deepest Look Into Space 6
The NTT Provides the Deepest Look Into Space 6. A. PETERSON, Mount Stromlo Observatory,Australian National University, Canberra S. D'ODORICO, M. TARENGHI and E. J. WAMPLER, ESO The ESO New Technology Telescope r on La Silla has again proven its extraor- - dinary abilities. It has now produced the "deepest" view into the distant regions of the Universe ever obtained with ground- or space-based telescopes. Figure 1 : This picture is a reproduction of a I.1 x 1.1 arcmin portion of a composite im- age of forty-one 10-minute exposures in the V band of a field at high galactic latitude in the constellation of Sextans (R.A. loh 45'7 Decl. -0' 143. The individual images were obtained with the EMMI imager/spectrograph at the Nas- myth focus of the ESO 3.5-m New Technolo- gy Telescope using a 1000 x 1000 pixel Thomson CCD. This combination gave a full field of 7.6 x 7.6 arcmin and a pixel size of 0.44 arcsec. The average seeing during these exposures was 1.0 arcsec. The telescope was offset between the indi- vidual exposures so that the sky background could be used to flat-field the frame. This procedure also removed the effects of cos- mic rays and blemishes in the CCD. More than 97% of the objects seen in this sub- field are galaxies. For the brighter galax- ies, there is good agreement between the galaxy counts of Tyson (1988, Astron. J., 96, 1) and the NTT counts for the brighter galax- ies. -
Young Globular Clusters and Dwarf Spheroidals
View metadata, citation and similar papers at core.ac.uk brought to you by CORE provided by CERN Document Server Young Globular Clusters and Dwarf Spheroidals Sidney van den Bergh Dominion Astrophysical Observatory Herzberg Institute of Astrophysics National Research Council of Canada 5071 West Saanich Road Victoria, British Columbia, V8X 4M6 Canada ABSTRACT Most of the globular clusters in the main body of the Galactic halo were formed almost simultaneously. However, globular cluster formation in dwarf spheroidal galaxies appears to have extended over a significant fraction of a Hubble time. This suggests that the factors which suppressed late-time formation of globulars in the main body of the Galactic halo were not operative in dwarf spheroidal galaxies. Possibly the presence of significant numbers of “young” globulars at RGC > 15 kpc can be accounted for by the assumption that many of these objects were formed in Sagittarius-like (but not Fornax-like) dwarf spheroidal galaxies, that were subsequently destroyed by Galactic tidal forces. It would be of interest to search for low-luminosity remnants of parental dwarf spheroidals around the “young” globulars Eridanus, Palomar 1, 3, 14, and Terzan 7. Furthermore multi-color photometry could be used to search for the remnants of the super-associations, within which outer halo globular clusters originally formed. Such envelopes are expected to have been tidally stripped from globulars in the inner halo. Subject headings: Globular clusters - galaxies: dwarf The galaxy is, in fact, nothing but a congeries of innumerable stars grouped together in clusters. Galileo (1610) –2– 1. Introduction The vast majority of Galactic globular clusters appear to have formed at about the same time (e.g. -
Globular Clusters in the Inner Galaxy Classified from Dynamical Orbital
MNRAS 000,1{17 (2019) Preprint 14 November 2019 Compiled using MNRAS LATEX style file v3.0 Globular clusters in the inner Galaxy classified from dynamical orbital criteria Angeles P´erez-Villegas,1? Beatriz Barbuy,1 Leandro Kerber,2 Sergio Ortolani3 Stefano O. Souza 1 and Eduardo Bica,4 1Universidade de S~aoPaulo, IAG, Rua do Mat~ao 1226, Cidade Universit´aria, S~ao Paulo 05508-900, Brazil 2Universidade Estadual de Santa Cruz, Rodovia Jorge Amado km 16, Ilh´eus 45662-000, Brazil 3Dipartimento di Fisica e Astronomia `Galileo Galilei', Universit`adi Padova, Vicolo dell'Osservatorio 3, Padova, I-35122, Italy 4Universidade Federal do Rio Grande do Sul, Departamento de Astronomia, CP 15051, Porto Alegre 91501-970, Brazil Accepted XXX. Received YYY; in original form ZZZ ABSTRACT Globular clusters (GCs) are the most ancient stellar systems in the Milky Way. There- fore, they play a key role in the understanding of the early chemical and dynamical evolution of our Galaxy. Around 40% of them are placed within ∼ 4 kpc from the Galactic center. In that region, all Galactic components overlap, making their disen- tanglement a challenging task. With Gaia DR2, we have accurate absolute proper mo- tions for the entire sample of known GCs that have been associated with the bulge/bar region. Combining them with distances, from RR Lyrae when available, as well as ra- dial velocities from spectroscopy, we can perform an orbital analysis of the sample, employing a steady Galactic potential with a bar. We applied a clustering algorithm to the orbital parameters apogalactic distance and the maximum vertical excursion from the plane, in order to identify the clusters that have high probability to belong to the bulge/bar, thick disk, inner halo, or outer halo component. -
Galactic Metal-Poor Halo E NCYCLOPEDIA of a STRONOMY and a STROPHYSICS
Galactic Metal-Poor Halo E NCYCLOPEDIA OF A STRONOMY AND A STROPHYSICS Galactic Metal-Poor Halo Most of the gas, stars and clusters in our Milky Way Galaxy are distributed in its rotating, metal-rich, gas-rich and flattened disk and in the more slowly rotating, metal- rich and gas-poor bulge. The Galaxy’s halo is roughly spheroidal in shape, and extends, with decreasing density, out to distances comparable with those of the Magellanic Clouds and the dwarf spheroidal galaxies that have been collected around the Galaxy. Aside from its roughly spheroidal distribution, the most salient general properties of the halo are its low metallicity relative to the bulk of the Galaxy’s stars, its lack of a gaseous counterpart, unlike the Galactic disk, and its great age. The kinematics of the stellar halo is closely coupled to the spheroidal distribution. Solar neighborhood disk stars move at a speed of about 220 km s−1 toward a point in the plane ◦ and 90 from the Galactic center. Stars belonging to the spheroidal halo do not share such ordered motion, and thus appear to have ‘high velocities’ relative to the Sun. Their orbital energies are often comparable with those of the disk stars but they are directed differently, often on orbits that have a smaller component of rotation or angular Figure 1. The distribution of [Fe/H] values for globular clusters. momentum. Following the original description by Baade in 1944, the disk stars are often called POPULATION I while still used to measure R . The recognizability of globular the metal-poor halo stars belong to POPULATION II. -
Assessing the Milky Way Satellites Associated with the Sagittarius Dwarf Spheroidal Galaxy
DRAFT: November 8, 2018 Preprint typeset using LATEX style emulateapj v. 11/10/09 ASSESSING THE MILKY WAY SATELLITES ASSOCIATED WITH THE SAGITTARIUS DWARF SPHEROIDAL GALAXY David R. Law1,2, Steven R. Majewski3 DRAFT: November 8, 2018 ABSTRACT Numerical models of the tidal disruption of the Sagittarius (Sgr) dwarf galaxy have recently been developed that for the first time simultaneously satisfy most observational constraints on the angular position, distance, and radial velocity trends of both leading and trailing tidal streams emanating from the dwarf. We use these dynamical models in combination with extant 3-D position and velocity data for Galactic globular clusters and dSph galaxies to identify those Milky Way satellites that are likely to have originally formed in the gravitational potential well of the Sgr dwarf, and have been stripped from Sgr during its extended interaction with the Milky Way. We conclude that the globular clusters Arp 2, M 54, NGC 5634, Terzan 8, and Whiting 1 are almost certainly associated with the Sgr dwarf, and that Berkeley 29, NGC 5053, Pal 12, and Terzan 7 are likely to be as well (albeit at lower confidence). The initial Sgr system therefore may have contained 5-9 globular clusters, corresponding to a specific frequency SN =5−9 for an initial Sgr luminosity MV = −15.0. Our result is consistent with the 8±2 genuine Sgr globular clusters expected on the basis of statistical modeling of the Galactic globular cluster distribution and the corresponding false-association rate due to chance alignments with the Sgr streams. The globular clusters identified as most likely to be associated with Sgr are consistent with previous reconstructions of the Sgr age-metallicity relation, and show no evidence for a second- parameter effect shaping their horizontal branch morphologies. -
Neutron-Capture Elements in Dwarf Galaxies III
Neutron-capture elements in dwarf galaxies III. A homogenized analysis of 13 dwarf spheroidal and ultra-faint galaxies Reichert, M.; Hansen, C. J.; Hanke, M.; Skuladottir, A.; Arcones, A.; Grebel, E. K. Published in: Astronomy & Astrophysics DOI: 10.1051/0004-6361/201936930 Publication date: 2020 Document version Publisher's PDF, also known as Version of record Document license: CC BY-NC Citation for published version (APA): Reichert, M., Hansen, C. J., Hanke, M., Skuladottir, A., Arcones, A., & Grebel, E. K. (2020). Neutron-capture elements in dwarf galaxies III. A homogenized analysis of 13 dwarf spheroidal and ultra-faint galaxies. Astronomy & Astrophysics, 641, [A127]. https://doi.org/10.1051/0004-6361/201936930 Download date: 09. Oct. 2021 A&A 641, A127 (2020) Astronomy https://doi.org/10.1051/0004-6361/201936930 & c ESO 2020 Astrophysics Neutron-capture elements in dwarf galaxies III. A homogenized analysis of 13 dwarf spheroidal and ultra-faint galaxies?,?? M. Reichert1, C. J. Hansen2,3, M. Hanke4, Á. Skúladóttir2,5,6 , A. Arcones1,7, and E. K. Grebel4 1 Technische Universität Darmstadt, Institut für Kernphysik, Schlossgartenstr. 2, 64289 Darmstadt, Germany e-mail: [email protected] 2 Max-Planck-Institut für Astronomie, Königstuhl 17, 69117 Heidelberg, Germany e-mail: [email protected] 3 Copenhagen University, Dark Cosmology Centre, The Niels Bohr Institute, Vibenshuset, Lyngbyvej 2, 2100 Copenhagen, Denmark 4 Astronomisches Rechen-Institut, Zentrum für Astronomie der Universität Heidelberg, Mönchhofstr. 12-14, 69120 Heidelberg, Germany 5 Dipartimento di Fisica e Astronomia, Universitá degli Studi di Firenze, Via G. Sansone 1, 50019 Sesto Fiorentino, Italy 6 INAF/Osservatorio Astrofisico di Arcetri, Largo E. -
108 Afocal Procedure, 105 Age of Globular Clusters, 25, 28–29 O
Index Index Achromats, 70, 73, 79 Apochromats (APO), 70, Averted vision Adhafera, 44 73, 79 technique, 96, 98, Adobe Photoshop Aquarius, 43, 99 112 (software), 108 Aquila, 10, 36, 45, 65 Afocal procedure, 105 Arches cluster, 23 B1620-26, 37 Age Archinal, Brent, 63, 64, Barkhatova (Bar) of globular clusters, 89, 195 catalogue, 196 25, 28–29 Arcturus, 43 Barlow lens, 78–79, 110 of open clusters, Aricebo radio telescope, Barnard’s Galaxy, 49 15–16 33 Basel (Bas) catalogue, 196 of star complexes, 41 Aries, 45 Bayer classification of stellar associations, Arp 2, 51 system, 93 39, 41–42 Arp catalogue, 197 Be16, 63 of the universe, 28 Arp-Madore (AM)-1, 33 Beehive Cluster, 13, 60, Aldebaran, 43 Arp-Madore (AM)-2, 148 Alessi, 22, 61 48, 65 Bergeron 1, 22 Alessi catalogue, 196 Arp-Madore (AM) Bergeron, J., 22 Algenubi, 44 catalogue, 197 Berkeley 11, 124f, 125 Algieba, 44 Asterisms, 43–45, Berkeley 17, 15 Algol (Demon Star), 65, 94 Berkeley 19, 130 21 Astronomy (magazine), Berkeley 29, 18 Alnilam, 5–6 89 Berkeley 42, 171–173 Alnitak, 5–6 Astronomy Now Berkeley (Be) catalogue, Alpha Centauri, 25 (magazine), 89 196 Alpha Orionis, 93 Astrophotography, 94, Beta Pictoris, 42 Alpha Persei, 40 101, 102–103 Beta Piscium, 44 Altair, 44 Astroplanner (software), Betelgeuse, 93 Alterf, 44 90 Big Bang, 5, 29 Altitude-Azimuth Astro-Snap (software), Big Dipper, 19, 43 (Alt-Az) mount, 107 Binary millisecond 75–76 AstroStack (software), pulsars, 30 Andromeda Galaxy, 36, 108 Binary stars, 8, 52 39, 41, 48, 52, 61 AstroVideo (software), in globular clusters, ANR 1947 -
The ACS Survey of Galactic Globular Clusters. Xi. the Three- Dimensional Orientation of the Sagittarius Dwarf Spheroidal Galaxy and Its Globular Clusters
Dartmouth College Dartmouth Digital Commons Dartmouth Scholarship Faculty Work 12-10-2011 The ACS Survey of Galactic Globular Clusters. Xi. The Three- Dimensional Orientation of the Sagittarius Dwarf Spheroidal Galaxy and its Globular Clusters Michael H. H. Siegel The Pennsylvania State University Steven R. Majewski University of Virginia David R. Law University of California, Los Angeles Ata Sarajedini University of Florida Aaron Dotter University of Victoria FSeeollow next this page and for additional additional works authors at: https:/ /digitalcommons.dartmouth.edu/facoa Part of the External Galaxies Commons, and the Stars, Interstellar Medium and the Galaxy Commons Dartmouth Digital Commons Citation Siegel, Michael H. H.; Majewski, Steven R.; Law, David R.; Sarajedini, Ata; Dotter, Aaron; Marín-Franch, A; and Chaboyer, Brian, "The ACS Survey of Galactic Globular Clusters. Xi. The Three-Dimensional Orientation of the Sagittarius Dwarf Spheroidal Galaxy and its Globular Clusters" (2011). Dartmouth Scholarship. 2205. https://digitalcommons.dartmouth.edu/facoa/2205 This Article is brought to you for free and open access by the Faculty Work at Dartmouth Digital Commons. It has been accepted for inclusion in Dartmouth Scholarship by an authorized administrator of Dartmouth Digital Commons. For more information, please contact [email protected]. Authors Michael H. H. Siegel, Steven R. Majewski, David R. Law, Ata Sarajedini, Aaron Dotter, A Marín-Franch, and Brian Chaboyer This article is available at Dartmouth Digital Commons: https://digitalcommons.dartmouth.edu/facoa/2205 The Astrophysical Journal, 743:20 (16pp), 2011 December 10 doi:10.1088/0004-637X/743/1/20 C 2011. The American Astronomical Society. All rights reserved. Printed in the U.S.A. -
Chemical Abundances in Terzan 7 ?
Mem. S.A.It. Vol. 75, 396 c SAIt 2004 Memorie della Chemical abundances in Terzan 7 ? L. Sbordone1;2, P. Bonifacio3, G. Marconi1;4, and R. Buonanno2;4 1 European Southern Observatory – Chile e-mail: [email protected] 2 Dipartimento di Fisica, Universita´ di Roma 2 “Tor Vergata” 3 Istituto Nazionale di Astrofisica - Osservatorio Astronomico di Trieste 4 Istituto Nazionale di Astrofisica - Osservatorio Astronomico di Roma Abstract. We present abundances for Mg, Si, Ca, Fe, and Ni for 3 giants in the sparse globular cluster Terzan 7, physically associated with the Sagittarius dwarf Spheroidal galaxy (Sgr dSph), which is presently being tidally disrupted by the Milky Way. The data, obtained with VLT-UVES, show a mean [Fe/H]= −0:57, a solar-scaled α content ([α/Fe]∼0) and a significant Ni underabundance ([Ni/Fe]= −0:2). This enforces Ter 7’s membership to the Sgr dSph system, which shows a similar chemical “signature”. Key words. Globular clusters: individual: Terzan 7 – galaxies: individual: Sgr dSph – stars: abundances – galaxies: abundances 1. Introduction S/N ∼ 30 at 580 nm. Equivalent widths (EWs) have been measured for lines of Fe I, Fe II, Mg The globular cluster Terzan 7 (Buonanno et al. I, Si I, Ca I and Ni I. The effective temperatures 1995) is a small, young and metal rich glob- have been derived from (V − I)0 colors by us- ular, physically associated with the Sgr dSph ing the calibration of Alonso et al. (1999). The (Marconi et al. 1998). The closest neighbour analysis was performed with codes ATLAS9, of the Milky Way, the Sgr dSph is presently WIDTH and SYNTHE (Kurucz 1993). -
Globular Clusters 1
Globular Clusters 1 www.FaintFuzzies.com Globular Clusters 2 www.FaintFuzzies.com Globular Clusters (Includes all known globulars in the Milky Way above declination of -50º plus some extras) by Alvin Huey www.faintfuzzies.com Last updated: March 27, 2014 Globular Clusters 3 www.FaintFuzzies.com Other books by Alvin H. Huey Hickson Group Observer’s Guide The Abell Planetary Observer’s Guide Observing the Arp Peculiar Galaxies Downloadable Guides by FaintFuzzies.com The Local Group Selected Small Galaxy Groups Galaxy Trios and Triple Systems Selected Shakhbazian Groups Globular Clusters Observing Planetary Nebulae and Supernovae Remnants Observing the Abell Galaxy Clusters The Rose Catalogue of Compact Galaxies Flat Galaxies Ring Galaxies Variable Galaxies The Voronstov-Velyaminov Catalogue – Part I and II Object of the Week 2012 and 2013 – Deep Sky Forum Copyright © 2008 – 2014 by Alvin Huey www.faintfuzzies.com All rights reserved Copyright granted to individuals to make single copies of works for private, personal and non-commercial purposes All Maps by MegaStarTM v5 All DSS images (Digital Sky Survey) http://archive.stsci.edu/dss/acknowledging.html This and other publications by the author are available through www.faintfuzzies.com Globular Clusters 4 www.FaintFuzzies.com Table of Contents Globular Cluster Index ........................................................................ 6 How to Use the Atlas ........................................................................ 10 The Milky Way Globular Clusters ....................................................