Syntheses of Enantiomerically Pure Chiral Hosts Using Amino Acid Derived Ligands with Fe(III), Ni(II) and Cu(II) Ions
Total Page:16
File Type:pdf, Size:1020Kb
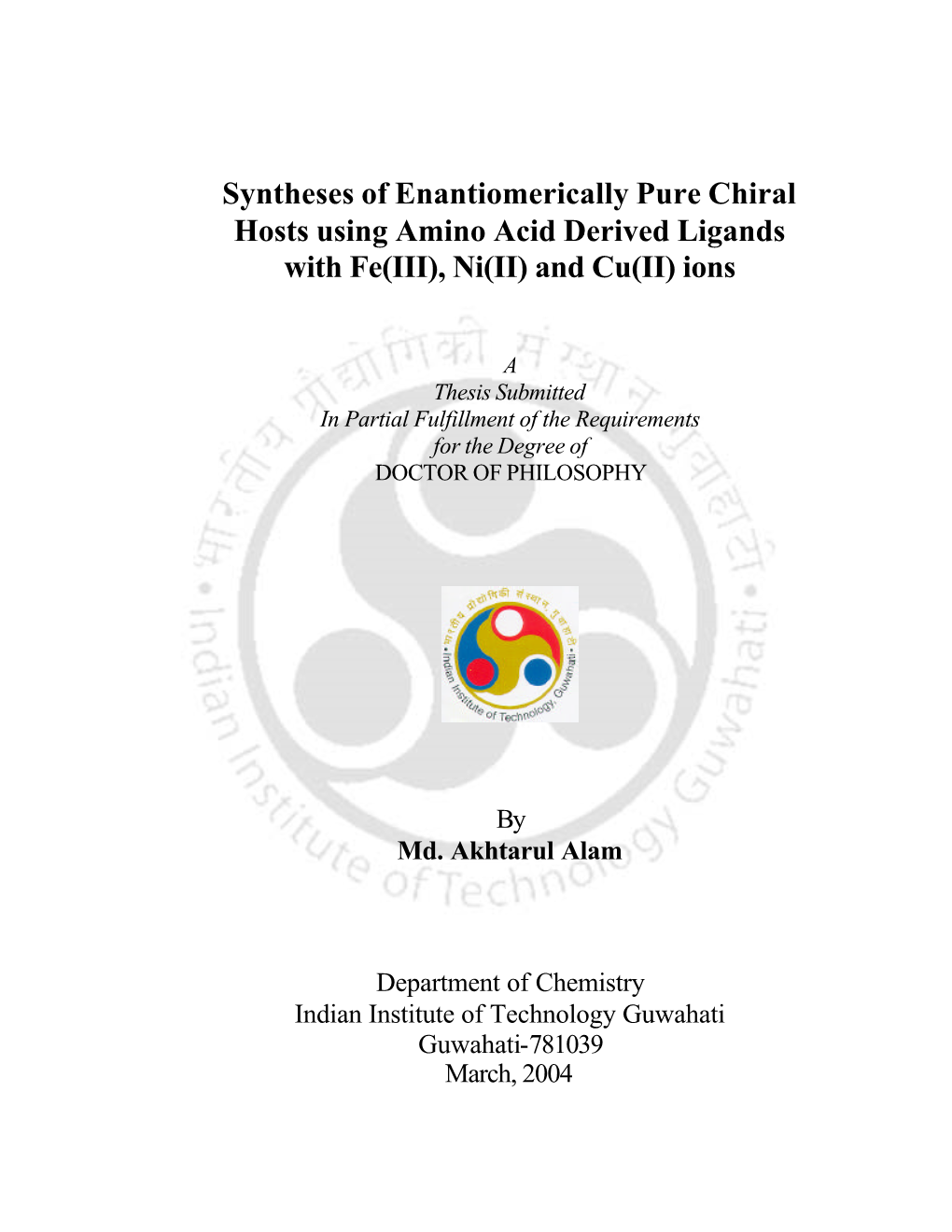
Load more
Recommended publications
-
Optimization of Chiral Separation of Nadolol by Simulated Moving Bed Technology
Western University Scholarship@Western Electronic Thesis and Dissertation Repository 11-30-2012 12:00 AM Optimization of Chiral Separation of Nadolol by Simulated Moving Bed Technology Nesma Nehad Hashem The University of Western Ontario Supervisor Dr. Ajay Ray The University of Western Ontario Joint Supervisor Dr. Hassan Gomaa The University of Western Ontario Graduate Program in Chemical and Biochemical Engineering A thesis submitted in partial fulfillment of the equirr ements for the degree in Master of Engineering Science © Nesma Nehad Hashem 2012 Follow this and additional works at: https://ir.lib.uwo.ca/etd Part of the Chemical Engineering Commons, Chemicals and Drugs Commons, and the Physical Sciences and Mathematics Commons Recommended Citation Hashem, Nesma Nehad, "Optimization of Chiral Separation of Nadolol by Simulated Moving Bed Technology" (2012). Electronic Thesis and Dissertation Repository. 973. https://ir.lib.uwo.ca/etd/973 This Dissertation/Thesis is brought to you for free and open access by Scholarship@Western. It has been accepted for inclusion in Electronic Thesis and Dissertation Repository by an authorized administrator of Scholarship@Western. For more information, please contact [email protected]. OPTIMIZATION OF CHIRAL SEPARATION OF NADOLOL BY SIMULATED MOVING BED TECHNOLOGY (Spine title: Optimization of enantioseparation of Nadolol by SMB) (Thesis format: Monograph) By Nesma Nehad Hashem Graduate Program in Chemical and Biochemical Engineering A thesis submitted in partial fulfillment of the requirements for the degree of Master of Engineering Science The School of Graduate and Postdoctoral Studies The University of Western Ontario London, Ontario, Canada © Nesma Nehad Hashem 2012 THE UNIVERSITY OF WESTERN ONTARIO SCHOOL OF GRADUATE AND POSTDOCTORAL STUDIES CERTIFICATE OF EXAMINATION Supervisor Examiners ______________________________ ______________________________ Dr. -
Medchem Russia 2019
Ural Branch of the Russian Academy of Sciences MedChem Russia 2019 4th Russian Conference on Medicinal Chemistry with international participants June 10-14, 2019 Ekaterinburg, Russia Abstract book © Ural Branch of the Russian Academy of Sciences. All rights reserved © Authors, 2019 The conference is held with the financial support of the Russian Foundation for Basic Research, project No. 19-03-20012 4th Russian Conference on Medicinal Chemistry with international participants. MedChem Russia 2019 Abstract book – Ekaterinburg : Ural Branch of the Russian Academy of Sciences, 2019. – 448 p. ISBN 978-5-7691-2521-8 Abstract book includes abstracts of plenary lectures, oral and poster presentations, and correspondent presentations of the Conference ORGANIZERS OF THE CONFERENCE Russian Academy of Sciences Ural Branch of the Russian Academy of Sciences Ministry of Science and Higher Education of the Russian Federation Ministry of Health of the Russian Federation Ural Federal University named after the First President of Russia B.N. Yeltsin Sverdlovsk Oblast Government Ministry of Industry and Science of Sverdlovsk Oblast Ekaterinburg City Administration Department of Chemistry and Material Sciences of RAS Scientific Council on Medical Chemistry of RAS Lomonosov Moscow State University, Faculty of Chemistry Postovsky Institute of Organic Synthesis of UBRAS Institute of Immunology and Physiology of UBRAS M.N. Mikheev Institute of Metal Physics of of UBRAS Institute of Physiologically Active Compounds of RAS N.N. Blokhin National Medical Research -
Enantiomeric Quantification of Psychoactive Substances and Beta Blockers by Gas Chromatography-Mass Spectrometry in Influents of Wastewater Treatment Plants
Enantiomeric quantification of psychoactive substances and beta blockers by gas chromatography-mass spectrometry in influents of wastewater treatment plants Ricardo Daniel Teixeira Gonçalves Dissertation of the 2nd Cycle of Studies Conducive to the Master’s Degree in Clinical and Forensic Analytical Toxicology, Faculty of Pharmacy, University of Porto Work performed under the orientation of: Professor Doctor Maria Elizabeth Tiritan Professor Doctor Cláudia Maria Rosa Ribeiro September 2018 IT IS NOT PERMITED TO REPRODUCE ANY PART OF THIS DISSERTATION DE ACORDO COM A LEGISLAÇÃO EM VIGOR, NÃO É PERMITIDA A REPRODUÇÃO DE QUALQUER PARTE DESTA DISSERTAÇÃO Agradecimentos Em primeiro lugar gostaria de agradecer às minhas orientadoras (ou mães da ciência), a Professora Doutora Maria Elizabeth Tiritan e a Professora Doutora Cláudia Ribeiro por me terem aceitado (adotado) para poder continuar este projeto. Obrigado por me ajudarem na iniciação de um projeto de raiz, que depois de quatro anos e muitas frustrações, vejo que não foi em vão. Por toda a ajuda, insistência, paciência, preocupação e compreensão que sempre tiveram, o maior obrigado! À Dra. Sara Cravo do laboratório de química orgânica da FFUP, que me recebeu de braços abertos, batalhou comigo durante meses e me deixou usar e abusar do seu laboratório de cromatografia gasosa. Obrigado por toda a ajuda, paciência, explicações, disponibilidade e acima de tudo, por me compreender nos momentos mais desesperantes. Estou-lhe profundamente grato por me fazer sentir “em casa” e por sempre arranjar soluções! Ao Professor Doutor Carlos Afonso do laboratório de química orgânica da FFUP por me autorizar a invadir o laboratório de química orgânica e pela preocupação constante com o progresso do trabalho. -
Pharmaceuticals Through the Looking Glass
Pharmaceuticals Through The Looking Glass. By Anita R. Maguire,Department of Chemistry, Analytical and Biological, Chemistry Research Facility, University College, Cork. This lecture will firstly give an overview of the pharmaceutical industry in Ireland, then outline how drugs are discovered and, in particular, how this process has changed in recent years, and finally focus on the significance of asymmetry in bioactive compounds used as pharmaceutical agents, illustrating this with some of our recent research in this area. The reference to the Lewis Carroll’s famous work Though the Looking Glass may seem obscure at the outset – is it simply a ploy to make a Chemistry lecture appear more attractive by raising curiosity in the audience? Or does it refer to the nature of scientific research where everything appears ‘curiouser and curiouser..’. By the end of the lecture the relevance should become clear. Pharmaceutical Industry in Ireland Globally the pharmaceutical sector is one of the most successful industrial sectors.1 It is distinguished from other industrial sectors by the very high investment of its sales on R&D, typically 15-20% of sales are reinvested in discovering and developing new drugs, much higher than the typical single digit investment in most sectors. The reason for this is very simple – discovering and developing new drugs is an extremely resource intensive activity, requiring large numbers of highly skilled staff, state-of- the-art equipment and facilities, and involves high risk to a company who invest enormous amounts in a drug which may fail at the final hurdle. High standards required by regulatory authorities to ensure the safety of pharmaceuticals require costly and lengthy studies into efficacy, toxicity etc. -
How to Obtain Enantiopure Drug Substance Through Crystallization?
How to obtain enantiopure drug substance through crystallization? Chirality is defined as the geometric property of an object, like a molecule, of not being superimposable with its mirror image. The two mirror images of a chiral molecule are called enantiomers. Enantiomers have essentially identical physical and chemical properties. However, in chiral environments, such as the receptors and enzymes in the body, enantiomers may behave differently and therefore exhibit different pharmacokinetic properties and exert quantitatively or qualitatively different pharmacological or toxicological effects. Chemical synthesis of chiral molecules usually delivers a racemic mixture, i.e. a 50:50 mixture of two enantiomers. In the past, chiral drugs were usually developed as racemic mixtures since separation into the individual enantiomers was technically difficult to achieve. However, technological advances have opened up the possibility of producing single enantiomers on large scale. Since the development of racemic mixtures raises issues of acceptable manufacturing control and adequate pharmacologic and toxicologic assessment, it has become common practice to develop chiral drugs as single enantiomers rather than as racemic mixtures. The development of procedures to obtain single enantiomers therefore constitute an important component of contemporary development programs for chiral drug substances. Essentially, enantiopure drug substance may be obtained in three ways, namely through separation, asymmetric synthesis or chiral crystallization. Chiral crystallization is an attractive approach in early development, as it is cost-effective, broadly applicable and easily scaled up. Chiral resolution through crystallization may be achieved in several ways. One technique is conversion of the enantiomeric substance into a diastereomeric salt (diastereomeric resolution). Diastereomers are substances that contain more than one chiral center and are not mirror images of one another. -
Development of Chromatographic Methods and Their Applications for the Analysis of Chiral Drug Metabolism by Enzymes Altered During Pregnancy
Research Collection Doctoral Thesis Development of chromatographic methods and their applications for the analysis of chiral drug metabolism by enzymes altered during pregnancy Author(s): Korner-Wyss, Sara Publication Date: 2015 Permanent Link: https://doi.org/10.3929/ethz-a-010361935 Rights / License: In Copyright - Non-Commercial Use Permitted This page was generated automatically upon download from the ETH Zurich Research Collection. For more information please consult the Terms of use. ETH Library DISS. ETH NO. 22444 DEVELOPMENT OF CHROMATOGRAPHIC METHODS AND THEIR APPLICATIONS FOR THE ANALYSIS OF CHIRAL DRUG METABOLISM BY ENZYMES ALTERED DURING PREGNANCY A thesis submitted to attain the degree of DOCTOR OF SCIENCES of ETH Zurich (Dr. sc. ETH Zurich) presented by SARA KORNER-WYSS Msc ETH Pharm. Sc, ETH Zurich born on 25.02.1981 citizen of Bern accepted on the recommendation of Prof. Dr. Karl-Heinz Altmann, examiner Prof. Dr. Ursula Quitterer, co-examiner Dr. Irmgard A. Werner, co-examiner 2015 An expert is a person who has made all the mistakes that can be made in a very narrow field. Niels Bohr (1885-1962) Dank Ich bedanke mich herzlich bei allen, die mich in den letzten Jahren unterst¨utzthaben und zum Gelingen dieser Arbeit beigetragen haben. Ganz besonders bedanke ich mich bei Herrn Prof. Karl-Heinz Altmann f¨urdas Vertrauen und die M¨oglichkeit unter seiner Leitung zu promovieren. Frau Prof. Ur- sula Quitterer bin ich sehr dankbar, dass sie bereit war das Korreferat f¨urdiese Arbeit zu ¨ubernehmen. Frau Dr. Irmgard A. Werner danke ich herzlich, dass sie mir die M¨oglichkeit gegeben hat in ihrer Gruppe die Doktorarbeit zu machen und sie in mir die Faszination zur Gaschromatographie wecken konnte. -
Table of Contents
I Synthesis and Evaluation of2,3-Dihydroquinazolinones as Dual Inhibitors of Angiogenesis and Cancer Cell Proliferation Gary Michael Chinigo Monroe, Connecticut B. S., University of Michigan at Ann Arbor, 2000 A Disseltation Presented to the Graduate Faculty of the University of Virginia in Candidacy for the Degree of Doctor of Philosophy Department of Chemistry University of Virginia January 2007 II © Copyright by Gary Michael Chinigo All Rights Reserved January 2007 III Abstract Dual inhibitors of cancer cell proliferation and angiogenesis have recently shown remarkable potential for the clinical treatment of several cancers. Inspired by this success, we have employed traditional medicinal chemistry techniques to develop a 2,3-dihydroquinazolin-4- one lead molecule with promising anti-proliferative and anti-angiogenic activity. These molecules, which we originally derived from thalidomide, have evolved into an extremely effective (sub-nanomolar) prospective drug candidate for the treatment of cancer. Described herein is an account of the many structural modifications made to this lead compound along with the corresponding effects on competitive 3H colchicine displacement from tubulin, microtubule depolymerization, and cytotoxicity toward several human cancer and endothelial cell lines. From these evaluations we were able to design 3rd generation analogs with significantly enhanced potency. Subsequent animal testing suggests these molecules are relatively non-toxic, bio-available, and efficacious at treating tumors in vivo – evidence which supports the possibility of our most active analogs being evaluated clinically. In addition to the SAR studies, we were compelled to develop synthetic methods enabling us to synthesize the enantiomers of these molecules. Exploration of several different approaches eventually led us to a chiral auxiliary based method of synthesis. -
Co-Crystallization Induced Spontaneous Deracemization
I UNIVERSITÉ CATHOLIQUE DE LOUVAIN INSTITUTE OF CONDENSED MATTER AND NANOSCIENCES (IMCN) Laboratory of Crystal Engineering Prof. Tom Leyssens Laboratory of Organic Chemistry Prof. Olivier Riant Development and Optimization of a new general thermodynamic deracemization method: Co-crystallization Induced Spontaneous Deracemization Thèse présentée en vue de l'obtention du grade de docteur en Sciences par Michael Guillot Louvain-la-Neuve Septembre 2020 II Jury Members Prof. Y. Cartigny Université de Rouen Normandie Prof. S. Kuhn Katholieke Universiteit Leuven Prof. M. Singleton Université catholique de Louvain Dr. K. Robeyns Université catholique de Louvain Prof. Y. Garcia (President) Université catholique de Louvain Prof. T. Leyssens (Promotor) Université catholique de Louvain Prof. O. Riant (Co-promotor) Université catholique de Louvain III Acknowledgements Je tiens tout d’abord à remercier mon promoteur, le professeur Tom Leyssens de m’avoir offert la chance de faire partie de son laboratoire pendant ces 4 années qui furent épanouissantes sur tous les plans. Je le remercie pour son encadrement tout au long de ma thèse, pour son soutien tant scientifique que personnel (surtout pendant « the dark year » où plus rien ne semblait marcher, je pense que tu vois de quel moment je parle Tom ;)) et pour la proximité qu’il sait créer au sein de son équipe. Je le remercie pour les congrès et séjours scientifiques que j’ai pu réaliser grâce à lui et qui ont tant participé à mon apprentissage autant scientifique que personnel. Je tiens tout aussi à remercier mon co-promoteur, le professeur Olivier Riant, pour ses conseils et corrections tout au long de ces quatres années de thèse. -
Stereopharmacology Research in Anaesthesiology
COPYRIGHT AND USE OF THIS THESIS This thesis must be used in accordance with the provisions of the Copyright Act 1968. Reproduction of material protected by copyright may be an infringement of copyright and copyright owners may be entitled to take legal action against persons who infringe their copyright. Section 51 (2) of the Copyright Act permits an authorized officer of a university library or archives to provide a copy (by communication or otherwise) of an unpublished thesis kept in the library or archives, to a person who satisfies the authorized officer that he or she requires the reproduction for the purposes of research or study. The Copyright Act grants the creator of a work a number of moral rights, specifically the right of attribution, the right against false attribution and the right of integrity. You may infringe the author’s moral rights if you: - fail to acknowledge the author of this thesis if you quote sections from the work - attribute this thesis to another author - subject this thesis to derogatory treatment which may prejudice the author’s reputation For further information contact the University’s Copyright Service. sydney.edu.au/copyright Stereopharmacological research in anaesthesiology A thesis based on selected published works submitted in fulfilment of the requirements for the degree of Doctor of Medical Science of Sydney Medical School The University of Sydney by Laurence Edward Mather PhD, MSc, BSc ( NSW ), DipApplChem ( SydTechColl ), FANZCA, FRCA, FFPMANZCA (Hon) July 2015 “It will be at once admitted that the medical practitioner ought to be acquainted with the strength of the various compounds which he applies as remedial agents, and that he ought, if possible, to be able to regulate their potency.” (John Snow, On the inhalation of the vapour of ether. -
Rivatives for Β-Blockers Practolol, Pindolol and Carteolol
Preprints (www.preprints.org) | NOT PEER-REVIEWED | Posted: 4 March 2021 Article Lipase Catalysed Synthesis of Enantiopure precursors and de- rivatives for β-Blockers Practolol, Pindolol and Carteolol. Morten A. Gundersen a, Guro Buaas Austli a, Sigrid Sløgedal Løvland, Mari Bergan Hansen, Mari Rødseth a and Elisabeth Egholm Jacobsen a, * a Department of chemistry, Norwegian University of Science and Technology, Høgskoleringen 5, 7491 Trond- heim * [email protected] Abstract: The -blocker (S)-practolol ((S)-N-(4-(2-hydroxy(isopropylamino)propoxy)phenyl)acet- amide) was synthesized with 96% enantiomeric excess (ee) from the chlorohydrin (R)-N-(4-(3- chloro-2 hydroxypropoxy)phenyl)acetamide, which was produced in 97% ee and with 27% yield. Racemic building block 1-((1H-indol-4-yl)oxy)-3-chloropropan-2-ol for the -blocker pindolol was produced in 53% yield and (R)-1-((1H-indol-4-yl)oxy)-3-chloropropan-2-ol was produced in 92% ee. The chlorohydrin 7-(3-chloro-2-hydroxypropoxy)-3,4-dihydroquinolin-2(1H)-one, a building block for a derivative of carteolol was produced in 77% yield. (R)-7-(3-chloro-2-hydroxypropoxy)-3,4-di- hydroquinolin-2(1H)-one was obtained in 96% ee. The S-enantiomer of this carteolol derivative was produced in 97% ee in 87% yield. Racemic building block 5-(3-chloro-2-hydroxypropoxy)- 3,4-dihy- droquinolin-2(1H)-one, building block for the drug carteolol was also produced in 53% yield, with 99% ee of the R-chlorohydrin (R)-5-(3-chloro-2-hydroxypropoxy)-3,4-dihydroquinolin-2(1H)-one. The yield of all four chlorohydrins increased by use of catalytic amounts of base. -
Fabrication Industrielle De Principes Actifs Pharmaceutiques Par
Fabrication industrielle de principes actifs pharmaceutiques par séparation chirale et racémisation : compétitivité mondiale par rapport aux voies de synthèse énantiosélective Fanny Sardou To cite this version: Fanny Sardou. Fabrication industrielle de principes actifs pharmaceutiques par séparation chirale et racémisation : compétitivité mondiale par rapport aux voies de synthèse énantiosélective. Sciences pharmaceutiques. 2014. hal-01732399 HAL Id: hal-01732399 https://hal.univ-lorraine.fr/hal-01732399 Submitted on 14 Mar 2018 HAL is a multi-disciplinary open access L’archive ouverte pluridisciplinaire HAL, est archive for the deposit and dissemination of sci- destinée au dépôt et à la diffusion de documents entific research documents, whether they are pub- scientifiques de niveau recherche, publiés ou non, lished or not. The documents may come from émanant des établissements d’enseignement et de teaching and research institutions in France or recherche français ou étrangers, des laboratoires abroad, or from public or private research centers. publics ou privés. AVERTISSEMENT Ce document est le fruit d'un long travail approuvé par le jury de soutenance et mis à disposition de l'ensemble de la communauté universitaire élargie. Il est soumis à la propriété intellectuelle de l'auteur. Ceci implique une obligation de citation et de référencement lors de l’utilisation de ce document. D'autre part, toute contrefaçon, plagiat, reproduction illicite encourt une poursuite pénale. Contact : [email protected] LIENS Code -
Effective Learning with Heterogenous, Noisy, Multi-Relational
EFFECTIVE LEARNING WITH HETEROGENOUS, NOISY, MULTI-RELATIONAL HEALTHCARE DATA by Devendra Singh Dhami APPROVED BY SUPERVISORY COMMITTEE: Sriraam Natarajan, Chair Vibhav Gogate Nicholas Ruozzi Gautam Kunapuli David Page Copyright c 2020 Devendra Singh Dhami All rights reserved This dissertation is dedicated to my parents and my wife who have been the pillars of strength throughout my ardous journey of realizing an important dream EFFECTIVE LEARNING WITH HETEROGENOUS, NOISY, MULTI-RELATIONAL HEALTHCARE DATA by DEVENDRA SINGH DHAMI, BE, MS DISSERTATION Presented to the Faculty of The University of Texas at Dallas in Partial Fulfillment of the Requirements for the Degree of DOCTOR OF PHILOSOPHY IN COMPUTER SCIENCE THE UNIVERSITY OF TEXAS AT DALLAS May 2020 ACKNOWLEDGMENTS First and foremost, I wish to convey my deepest gratitude and a very special thanks to my advisor, Dr. Sriraam Natarajan, for his unending support and guidance. He is the utmost reason that I got interested in the field of artificial intelligence and machine learning as well as research in general. My first brush with machine learning was his “Applied Machine Learning” class I took in 2014 in Indiana University and even now remains the favorite class of my educational career. His passion for teaching and research has been one of the foremost reasons for me pursuing a career in academia. The most important principle that I have learned from him is that “although having good papers is important, being a good scholar is more important”. He has also taught me to take failure in the correct spirit, to learn from it and finally convert the failure into success.