Development of Biocatalytic Approach Using Fungal Laccase
Total Page:16
File Type:pdf, Size:1020Kb
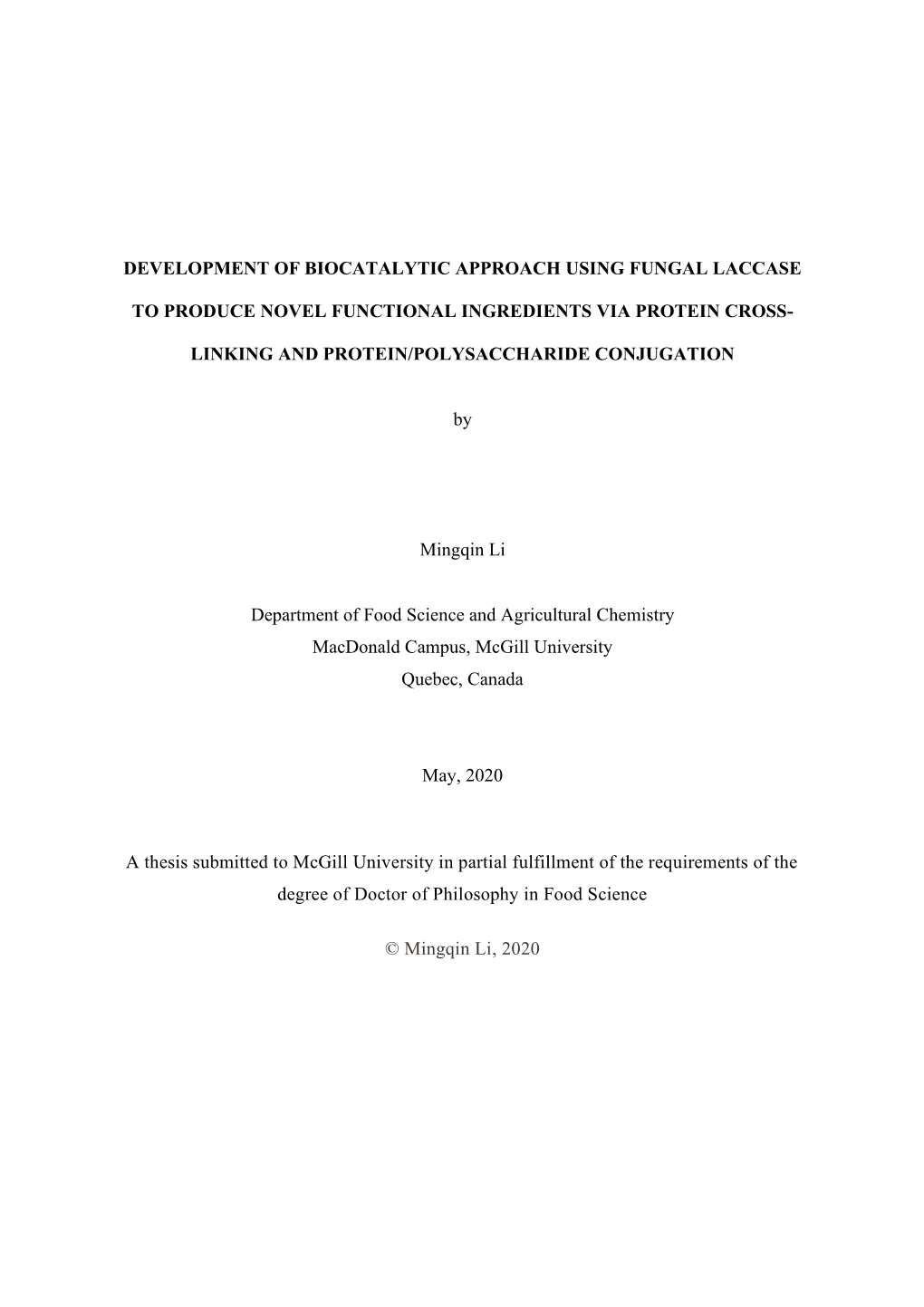
Load more
Recommended publications
-
Einfluss Von Lignin Und Ferulasäurederivaten Auf Die Adsorptionseigenschaften Von Ballaststoffen Und Den Fermentativen Abbau Durch Die Menschliche Darmflora
Einfluss von Lignin und Ferulasäurederivaten auf die Adsorptionseigenschaften von Ballaststoffen und den fermentativen Abbau durch die menschliche Darmflora Dissertation zur Erlangung des Doktorgrades des Fachbereiches Chemie der Universität Hamburg aus dem Institut für Biochemie und Lebensmittelchemie Abteilung für Lebensmittelchemie vorgelegt von Carola Funk aus Hamburg Hamburg 2007 Die vorliegende Arbeit wurde in der Zeit von April 2003 bis Oktober 2006 unter der Leitung von Herrn Prof. Dr. Dr. H. Steinhart am Institut für Biochemie und Lebensmittelchemie, Abtei- lung für Lebensmittelchemie, der Universität Hamburg angefertigt. 1. Gutachter: Prof. Dr. Dr. H. Steinhart 2. Gutachter: Prof. Dr. B. Bisping Tag der Disputation: 21.12.2007 Prüfungskommission: Prof. Dr. Dr. H. Steinhart Prof. Dr. E. Stahl-Biskup Dr. M. Körs Danksagung Zuallererst möchte ich mich herzlichst bei meinem Doktorvater Prof. Dr. Dr. Hans Steinhart für die Betreuung und Unterstützung meiner Doktorarbeit bedanken. Prof. Dr. Bernward Bisping danke ich für die bereitwillige Durchsicht meiner Arbeit als Zweit- gutachter. Mein ganz besonderer Dank gilt Prof. Dr. Mirko Bunzel, dem ich nicht nur das Thema meiner Doktorarbeit zu verdanken habe, sondern der mich und meine Arbeit auch maßgeblich ge- prägt hat. Ich habe durch ihn eine großartige wissenschaftliche Unterstützung erfahren. Ebenso schätze ich sehr seinen Humor und die Freundschaft, die sich im Laufe unserer ge- meinsamen Jahre an der Universität Hamburg entwickelt hat. Sehr dankbar bin ich ihm für die unvergesslichen USA-Forschungsaufenthalte, die er mir ermöglicht hat. Bei Ella Allerdings, Andreas Heinze, Reiko Yonekura und ganz besonders bei Diane Dob- berstein und Diana Gniechwitz bedanke ich mich für eine tolle gemeinsame Laborzeit. Es ist viel Wert, wenn im Labor nicht nur alle fachlichen Fragen diskutiert werden können, sondern die Arbeit ebenso mit so guten Freundschaften - auch über das Labor hinaus - eng verbun- den ist. -
Literature Review of Potential Health Benefits of Wild Rice and Wild Rice
Potential Health Benefits of Wild Rice and Wild Rice Products: Literature Review July 2012 By: Daniel D. Gallaher, Ph.D. (Principal Contact) Professor Department of Food Science and Nutrition University of Minnesota 612.624.0746, [email protected] 1334 Eckles Ave. St. Paul, MN 55108 Mirko Bunzel, Ph.D. Professor Chair of the Department of Food Chemistry and Phytochemistry Karlsruhe Institute of Technology – KIT Faculty of Chemistry and Biosciences, Institute of Applied Biosciences +49 721 608 42936, [email protected] TABLE OF CONTENTS INTRODUCTION ................................................................................................................................................................... 4 PHYTOCHEMICALS IN WILD RICE INCLUDING A LIST OF PHYTOCHEMICALS WITH POTENTIAL HEALTH BENEFITS ................. 4 DEFINITION OF PHYTOCHEMICALS .................................................................................................................................................... 4 PRIMARY METABOLITES FROM WILD RICE AFFECTING THE NUTRITIONAL QUALITY OF WILD RICE .................................................................... 5 Lipids .................................................................................................................................................................................. 5 Lipid content ..................................................................................................................................................................................... 5 Fatty -
Genotype and Environmental Influences on Grain Quality Characteristics of Australian Wheat Varieties
COPYRIGHT AND USE OF THIS THESIS This thesis must be used in accordance with the provisions of the Copyright Act 1968. Reproduction of material protected by copyright may be an infringement of copyright and copyright owners may be entitled to take legal action against persons who infringe their copyright. Section 51 (2) of the Copyright Act permits an authorized officer of a university library or archives to provide a copy (by communication or otherwise) of an unpublished thesis kept in the library or archives, to a person who satisfies the authorized officer that he or she requires the reproduction for the purposes of research or study. The Copyright Act grants the creator of a work a number of moral rights, specifically the right of attribution, the right against false attribution and the right of integrity. You may infringe the author’s moral rights if you: - fail to acknowledge the author of this thesis if you quote sections from the work - attribute this thesis to another author - subject this thesis to derogatory treatment which may prejudice the author’s reputation For further information contact the University’s Director of Copyright Services sydney.edu.au/copyright GENOTYPE AND ENVIRONMENTAL INFLUENCES ON GRAIN QUALITY CHARACTERISTICS OF AUSTRALIAN WHEAT VARIETIES Molook Al-Fadly A thesis submitted in fulfillment of the requirement of the degree of DOCTOR OF PHILOSOPHY (FOOD CHEMISTRY) Faculty of Agriculture and Environment, Plant and Food Sciences, The University of Sydney January 2014 I STATEMENT OF ORIGINALITY This thesis is submitted to the University of Sydney in fulfillment of the requirement for the degree of Doctor of Philosophy. -
Synthesis of Biomass-Containing Xylan Fragments and Evaluation of Ferulic Acid Esterase Activity
Downloaded from orbit.dtu.dk on: Oct 09, 2021 Synthesis of biomass-containing xylan fragments and evaluation of ferulic acid esterase activity Underlin, Emilie Nørmølle Publication date: 2018 Document Version Publisher's PDF, also known as Version of record Link back to DTU Orbit Citation (APA): Underlin, E. N. (2018). Synthesis of biomass-containing xylan fragments and evaluation of ferulic acid esterase activity. Technical University of Denmark. General rights Copyright and moral rights for the publications made accessible in the public portal are retained by the authors and/or other copyright owners and it is a condition of accessing publications that users recognise and abide by the legal requirements associated with these rights. Users may download and print one copy of any publication from the public portal for the purpose of private study or research. You may not further distribute the material or use it for any profit-making activity or commercial gain You may freely distribute the URL identifying the publication in the public portal If you believe that this document breaches copyright please contact us providing details, and we will remove access to the work immediately and investigate your claim. Synthesis of biomass-containing xylan fragments and evaluation of ferulic acid esterase activity Ph.D. thesis by Emilie Nørmølle Underlin, M.Sc. November 2018 DTU Chemistry ii Preface The work presented in this thesis encompasses the results obtained during my Ph.D. studies from December 2015 to November 2018 at the Department of Chemistry, Technical University of Denmark as part of the Danish program to obtain a Ph.D. -
Feruloyl Esterases for Biorefineries
fbioe-08-00332 April 21, 2020 Time: 14:37 # 1 ORIGINAL RESEARCH published: 23 April 2020 doi: 10.3389/fbioe.2020.00332 Feruloyl Esterases for Biorefineries: Subfamily Classified Specificity for Natural Substrates Emilie N. Underlin1,2†, Matthias Frommhagen1†, Adiphol Dilokpimol3, Gijs van Erven1, Ronald P. de Vries3 and Mirjam A. Kabel1* 1 Laboratory of Food Chemistry, Wageningen University & Research, Wageningen, Netherlands, 2 Department of Chemistry, Technical University of Denmark, Lyngby, Denmark, 3 Fungal Physiology, Westerdijk Fungal Biodiversity Institute and Fungal Molecular Physiology, Utrecht University, Utrecht, Netherlands Feruloyl esterases (FAEs) have an important role in the enzymatic conversion of lignocellulosic biomass by decoupling plant cell wall polysaccharides and lignin. Moreover, FAEs release anti-oxidative hydroxycinnamic acids (HCAs) from biomass. As a plethora of FAE candidates were found in fungal genomes, FAE classification related to substrate specificity is an indispensability for selection of most suitable Edited by: candidates. Hence, linking distinct substrate specificities to a FAE classification, such André Damasio, as the recently classified FAE subfamilies (SF), is a promising approach to improve Campinas State University, Brazil the application of these enzymes for a variety of industrial applications. In total, Reviewed by: Yasser Gaber, 14 FAEs that are classified members of SF1, 5, 6, 7, 9, and 13 were tested in this Beni-Suef University, Egypt research. All FAEs were investigated for their activity toward a variety of substrates: Noha M. Mesbah, Suez Canal University, Egypt synthetic model substrates, plant cell wall-derived substrates, including lignin, and Marcelo Vizoná Liberato, natural substrates. Released HCAs were determined using reverse phase-ultra high Brazilian Bioethanol Science and performance liquid chromatography coupled to UV detection and mass spectrometry. -
Phd Thesis Final Take 1
Downloaded from orbit.dtu.dk on: Sep 24, 2021 Enzymatic hydrolysis of corn bran arabinoxylan - theory versus practice Agger, Jane Publication date: 2011 Document Version Publisher's PDF, also known as Version of record Link back to DTU Orbit Citation (APA): Agger, J. (2011). Enzymatic hydrolysis of corn bran arabinoxylan: - theory versus practice. Technical University of Denmark. General rights Copyright and moral rights for the publications made accessible in the public portal are retained by the authors and/or other copyright owners and it is a condition of accessing publications that users recognise and abide by the legal requirements associated with these rights. Users may download and print one copy of any publication from the public portal for the purpose of private study or research. You may not further distribute the material or use it for any profit-making activity or commercial gain You may freely distribute the URL identifying the publication in the public portal If you believe that this document breaches copyright please contact us providing details, and we will remove access to the work immediately and investigate your claim. Ph D Thesis Enzymatic hydrolysis of corn bran arabinoxylan - theory versus practice Jane Agger March 2011 Bioprocess Engineering Department of Chemical and Biochemical Engineering Technical University of Denmark Preface The work presented here has been conducted during my time as a PhD student at Department of Chemical and Biochemical Engineering in the group Bioprocess Engineering at the Technical University of Denmark, from March 2007 and until March 2011. The work has been carefully supervised by Professor Anne S. -
The Impact of Processing on Wheat Grain Components Associated with Health Benefits
The impact of processing on wheat grain components associated with health benefits Paola Tosi 1*, Alyssa Hidalgo2, Valerie Lullien-Pellerin 3* 1 School of Agriculture Policy and Development, University of Reading, UK 2 Department of Food, Environmental and Nutritional Sciences (DeFENS), University of Milan, Via Celoria 2, 20133 Milan, Italy 3 IATE, Univ. Montpellier, CIRAD, INRA, Montpellier SupAgro, Montpellier, France * [email protected] or [email protected] Abstract Wheat based foods, mainly in the form of bread and pasta, are staples in many countries around the world and as such contribute substantially to nutrient intake of human beings. The mature wheat grain is composed for over 70-75% of its dry weight of starch and for around 10-14 % of protein, which has led to the widely spread perception of wheat foods as providers mainly of energy and protein to the human diet. Nonetheless, whole grains are also an important source of dietary fibers, vitamins and minerals and contain notable levels of bioactive compounds of health benefit as for examples lignans, phenolic acids, alkylresorcinols, phytosterols, folates and tocols. Processing is a pre-requisite for using cereal grains as food and obtaining a safe to consume and appealing final product for the consumer. It can help reducing potential hazardous molecules as pesticides, mycotoxins and heavy metals and allows to obtain products with varied and unique properties. Indeed, most of the importance of wheat grain in the human diet is due to its versatility to be processed into various end products like flour, semolina, and other bakery products. -
Characterization of Cereal Arabinoxylans and Their Health Benefits
Characterization of Cereal Arabinoxylans and their Health Benefits By Lovemore Nkhata Malunga A Thesis submitted to the Faculty of Graduate Studies of The University of Manitoba In Partial fulfillment of the Requirements for the degree of DOCTOR OF PHILOSOPHY Department of Food Science University of Manitoba Winnipeg, Manitoba Copyright © 2016 by Lovemore Nkhata Malunga ii Acknowledgement Many thanks should go to my advisor Dr. Trust Beta for giving me the opportunity to pursue doctoral studies. I am very grateful for her never ending support, patience and guidance during my entire doctoral studies. I would like also to acknowledge support given by Dr. Peter Eck during glucose transport assays for which I am so grateful. Many thanks should also go to Dr. Marta Izydorczyk for guidance and technical support during isolation and characterization of arabinoxylans. A special thank you to my committee members Dr. James Friel and Dr. Peter Jones for their support and guidance during my research. I would like also to thank the Academic Enhancement Fund (AEF) and the Faculty of Graduate Studies for funding my studies through GETs program. This research was funded by the Natural Sciences and Engineering Research Council of Canada (NSERC) (Discovery Grant Program) and the Canada Foundation for Innovation (CFI-Leaders Opportunity Fund and CFI-New Opportunities Fund). Many thanks go to Cargill Limited for provision of wheat aleurone samples. I am also grateful to the financial support received from the American Association of Cereal Chemists International (AACCI) Foundation Graduate fellowship. Furthermore, I would to like to thank the staff and students of the Department of Food Science for creating an enabling environment to conduct my studies. -
Enzyme Catalyzed Oxidative Cross-Linking of Feruloylated Pectic Polysaccharides from Sugar Beet Kinetics and Rheology
Downloaded from orbit.dtu.dk on: Oct 06, 2021 Enzyme catalyzed oxidative cross-linking of feruloylated pectic polysaccharides from sugar beet Kinetics and rheology Abang Zaidel, Dayang Norulfairuz Publication date: 2012 Document Version Publisher's PDF, also known as Version of record Link back to DTU Orbit Citation (APA): Abang Zaidel, D. N. (2012). Enzyme catalyzed oxidative cross-linking of feruloylated pectic polysaccharides from sugar beet: Kinetics and rheology. Technical University of Denmark. General rights Copyright and moral rights for the publications made accessible in the public portal are retained by the authors and/or other copyright owners and it is a condition of accessing publications that users recognise and abide by the legal requirements associated with these rights. Users may download and print one copy of any publication from the public portal for the purpose of private study or research. You may not further distribute the material or use it for any profit-making activity or commercial gain You may freely distribute the URL identifying the publication in the public portal If you believe that this document breaches copyright please contact us providing details, and we will remove access to the work immediately and investigate your claim. Enzyme catalyzed oxidative cross‐linking of feruloylated pectic polysaccharides from sugar beet – Kinetics and rheology PhD Thesis Dayang Norulfairuz Abang Zaidel Enzyme catalyzed oxidative cross‐linking of feruloylated pectic polysaccharides from sugar beet – Kinetics and rheology This thesis was prepared by Dayang Norulfairuz Abang Zaidel Supervised by Professor Anne S. Meyer January 2012 Center for Bioprocess Engineering Department of Chemical and Biochemical Engineering Technical University of Denmark Enzyme catalyzed oxidative cross‐linking of feruloylated pectic polysaccharides from sugar beet – Kinetics and rheology PREFACE This thesis is submitted in fulfillment of the requirements for the Doctorate (PhD) degree at the Technical University of Denmark (DTU). -
Characterization of Indigestible Fiber in Diets Based On
CHARACTERIZATION OF INDIGESTIBLE FIBER IN DIETS BASED ON CORN AND SOYBEAN MEAL OR CORN, SOYBEAN MEAL, AND DISTILLER DRIED GRAINS WITH SOLUBLES AND IN FECES FROM PIGS FED THESE DIETS BY JEIMMY PAOLA LANCHEROS CASTANEDA THESIS Submitted in partial fulfillment of the requirements for the degree of Master of Science in Animal Sciences in the Graduate College of the University of Illinois at Urbana-Champaign, 2021 Urbana, Illinois Master’s Committee Professor Hans H. Stein Professor Maria Cattai de Godoy Professor Emeritus George C. Fahey, Jr ABSTRACT Three experiments were conducted to determine concentrations of non-starch polysaccharides, total dietary fiber and phenolic acids in a diet based on corn and soybean meal (SBM), and in a diet based on corn, SBM, and distiller dried grains with solubles (DDGS), as well as in feces from pigs fed these diets. The in-vitro disappearance of DM without and with inclusion of exogenous enzymes in both diets was also determined. In Exp. 1, total NSP and dietary fiber in diets based on corn and SBM or corn, SBM, and DDGS and in fecal samples from pigs fed these diets were quantified. The hypothesis that the concentration of NSP in the diet based on corn, SBM, and DDGS, and in feces from pigs fed this diet is greater than in the corn and SBM diet and in feces from pigs fed this diet, was tested. Concentration of total NSP in the diet containing corn, SBM, and DDGS was greater than in the diet based on corn and SBM. In contrast, the concentration of total NSP in the feces from pigs fed these diets was not different. -
Phenolics and Phenolic-Polysaccharide Linkages in Chinese Water Chestnut (Eleocharis Dulcis) Cell Walls
Phenolics and phenolic-polysaccharide linkages in Chinese water chestnut (Eleocharis dulcis) cell walls. Terri Grassby BSc Chemistry with Industrial Experience Submitted for the degree of Doctor of Philosophy University of East Anglia Institute of Food Research March 2008 © This copy of the thesis has been supplied on condition that anyone who consults it is understood to recognise that its copyright rests with the author and that no quotation from the thesis, nor any information derived therefrom, may be published without the author’s prior written consent. ABSTRACT Abstract: The main aim was to investigate the cell-wall cross-links in Chinese water chestnut (CWC), in particular ferulic-acid-containing phenolic-polysaccharide cross-links. The secondary aims were: to understand the gross composition of CWC cell walls from the parenchyma, epidermis and sub-epidermis tissues of the corm and the role of cell-wall composition in the plant’s physiology, and to determine whether CWC contained higher oligomers of ferulic acid. Cell-wall composition was investigated using a range of chemical analyses including alkali phenolic extraction and methylation analysis. Chemical and biochemical methods were evaluated for their ability to produce oligosaccharide fragments attached to ferulic acid species. Mild acid hydrolysis followed by column chromatography using Biogel P-2 was the method chosen. LC-MS was used to identify compounds of interest. The compositions of the epidermal tissues differed particularly in the proportions of lignin and cellulose present. The relative amounts and proportions of the phenolics varied considerably, possibly indicating their functions in the different tissues. A multitude of phenolics were detected, a number of which now have detailed UV information recorded about them. -
Dissertation Diane Dobberstein
Charakterisierung der Zellwandstrukturkomponenten von Maisrestpflanzen als mögliche Einflussfaktoren der ruminalen Zellwandabbaubarkeit Dissertation zur Erlangung des Doktorgrades des Departments Chemie der Fakultät für Mathematik, Informatik und Naturwissenschaften der Universität Hamburg aus dem Institut für Biochemie und Lebensmittelchemie - Abteilung für Lebensmittelchemie - vorgelegt von Diane Dobberstein aus Hamburg Hamburg 2009 Die vorliegende Arbeit wurde in der Zeit von Mai 2004 bis Oktober 2007 unter der Leitung von Herrn Prof. Dr. Dr. H. Steinhart am Institut für Biochemie und Lebensmittelchemie, Abteilung Lebensmittelchemie, der Universität Hamburg angefertigt. 1. Gutachter: Prof. Dr. Dr. H. Steinhart 2. Gutachter: Prof. Dr. B. Bisping Tag der Disputation: 28.07.2009 Begutachtende der Disputaion: Prof. Dr. Dr. H. Steinhart Prof. Dr. E. Stahl-Biskup Dr. A. Paschke DANKSAGUNGEN Herrn Prof. Dr. Dr. H. Steinhart und Herrn Prof. Dr. Mirko Bunzel möchte ich für die Bereitstellung des Themas und die Betreuung dieser Arbeit danken. Herrn Prof. Dr. B. Bisping danke ich für die Übernahme des Koreferats. Neben den Mitarbeitern der Abteilung Lebensmittelchemie, möchte ich besonders Herrn Prof. Dr. Mirko Bunzel sowie allen ehemaligen und derzeitigen Mitgliedern seiner Arbeitsgruppe, insbesondere Frau Dr. Carola Funk, Frau Dr. Diana Bunzel und Herrn Heinze, für die stete Unterstützung und vor allem auch für die schöne Zeit danken. Mein Dank gilt auch Herrn Christian Gillot, Frau Sabrina Ment, Frau Caroline Indorf, Frau Anne Freudenstein und Herrn Tim Lubinus, die mit ihrer tatkräftigen Unterstützung zum Gelingen dieser Arbeit beigetragen haben. Frau Dr. Diana Bunzel und Herrn Dr. Michael Wigotzki danke ich für die Durchsicht des Skriptes. Bei Herrn Prof. F.J. Schwarz und Frau Dr. F. Zeller von der Abteilung für Tierernährung der Technischen Universität München, bedanke ich mich für die gute Zusammenarbeit, die Bereitstellung der Untersuchungsmaterialien und die vielen angeregten Diskussionen.