Atmospheric Optical Phenomena Result from Visible Light Interacting with the Earth's Atmosphere and Aerosols
Total Page:16
File Type:pdf, Size:1020Kb
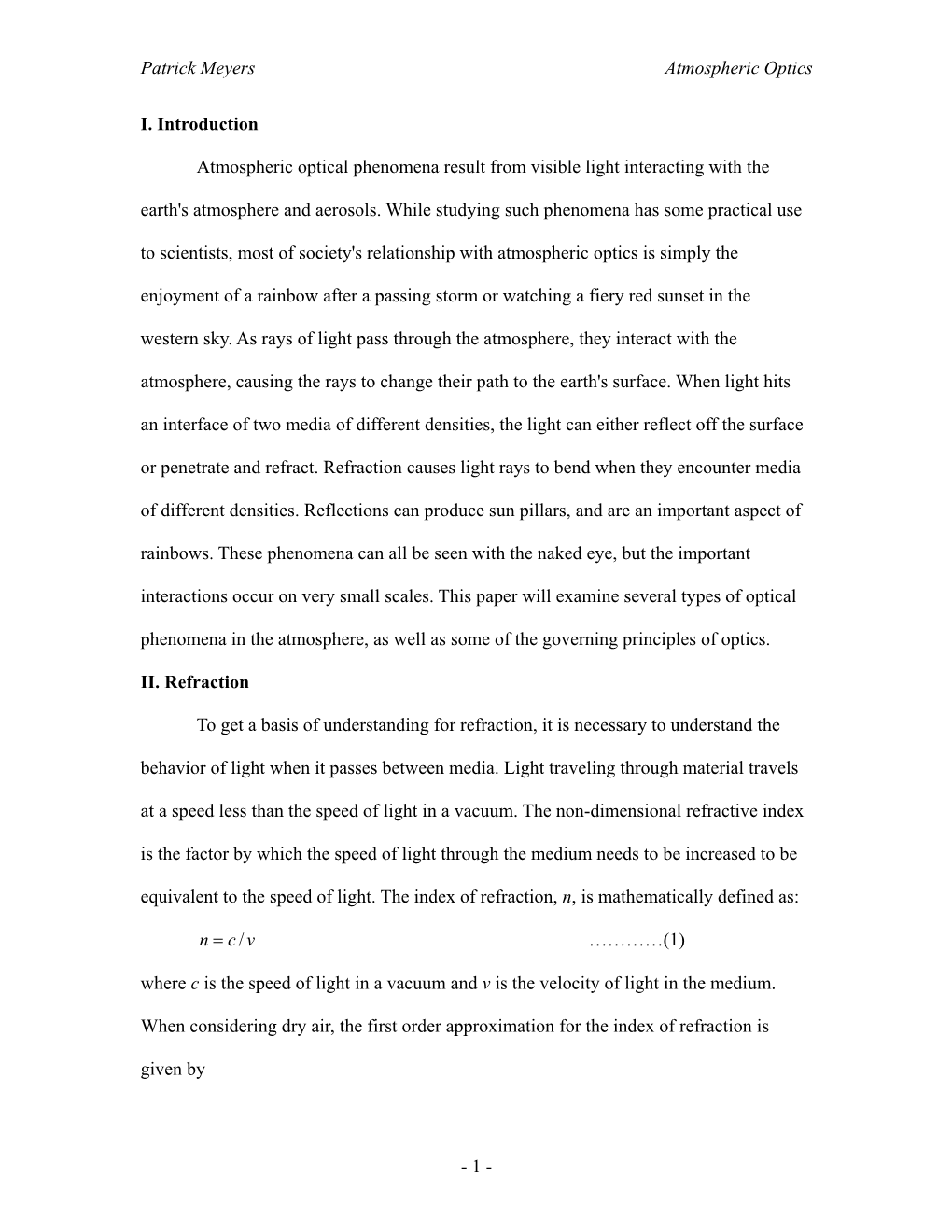
Load more
Recommended publications
-
Light Color and Atmospheric Light, Color, and Atmospheric Optics
Light, Color, and Atmospheric Optics Chapter 19 April 28 and 30, 2009 Colors • Sunlight contains all colors (visible spectrum) Wavelength Ranges in µm UV-C: 0.25 - 0.29 UV-B: 0.29 - 0.32 UV-A: 0.32 - 0.38 Visible: 0.38 - 0.75 Near IR: 0.75 - 4 Mid IR: 4 - 8 Longwave IR: 8 - 100 Colors • If object is white then all colors are reflected • If object is red then only red is reflected and all others are absorbed From Malm (1999). Introduction to Visibility Blue Skies and Hazy days • Selective scattering – Scattering depends on wavelength of light • Rayleigh scattering – Very small particles, gases • Mie scattering – Particles the size of wavelength of visible light • Blue haze • Some particles from plants: terpenes, α- pinene Blue Ridge Mountains • Blue haze caused by scattering of blue light by fine particles Creppyuscular Rays • Scattering of sunlight by dust and haze produces white bands Selective Scattering: Sunset • Notice the effects of scattering on color as sunlight penetrates into the atmosphere Twinkling, Twilight, and the Green Flash • Light that travels from a less dense to a more dense medium loses speed and bends toward the normal, while light that enters a less dense medium increase speed and bends away from the normal. • Apparent position, scintillation, and green flash all depend of density variations in the athtmosphere • Scintillation also indicates the amount of turbulence in the atmosphere Bending of Light Paths from Atmosphere Green Flash • Green flash at top may be seen under circumstances of very cold (dense) air near the surface rapidly changing to warmer (less dense) air aloft Mirages •Miraggyges are caused by refraction of light due to strong density differences in atmospheric layers usually caused by strong temperature differences. -
Photochemically Driven Collapse of Titan's Atmosphere
REPORTS has escaped, the surface temperature again Photochemically Driven Collapse decreases, down to about 86 K, slightly of Titan’s Atmosphere above the equilibrium temperature, because of the slight greenhouse effect resulting Ralph D. Lorenz,* Christopher P. McKay, Jonathan I. Lunine from the N2 opacity below (longward of) 200 cm21. Saturn’s giant moon Titan has a thick (1.5 bar) nitrogen atmosphere, which has a These calculations assume the present temperature structure that is controlled by the absorption of solar and thermal radiation solar constant of 15.6 W m22 and a surface by methane, hydrogen, and organic aerosols into which methane is irreversibly converted albedo of 0.2 and ignore the effects of N2 by photolysis. Previous studies of Titan’s climate evolution have been done with the condensation. As noted in earlier studies assumption that the methane abundance was maintained against photolytic depletion (5), when the atmosphere cools during the throughout Titan’s history, either by continuous supply from the interior or by buffering CH4 depletion, the model temperature at by a surface or near surface reservoir. Radiative-convective and radiative-saturated some altitudes in the atmosphere (here, at equilibrium models of Titan’s atmosphere show that methane depletion may have al- ;10 km for 20% CH4 surface humidity) lowed Titan’s atmosphere to cool so that nitrogen, its main constituent, condenses onto becomes lower than the saturation temper- the surface, collapsing Titan into a Triton-like frozen state with a thin atmosphere. -
Enhanced Total Internal Reflection Using Low-Index Nanolattice Materials
Letter Vol. 42, No. 20 / October 15 2017 / Optics Letters 4123 Enhanced total internal reflection using low-index nanolattice materials XU A. ZHANG,YI-AN CHEN,ABHIJEET BAGAL, AND CHIH-HAO CHANG* Department of Mechanical and Aerospace Engineering, North Carolina State University, Raleigh, North Carolina 27695, USA *Corresponding author: [email protected] Received 4 August 2017; revised 14 September 2017; accepted 16 September 2017; posted 19 September 2017 (Doc. ID 303972); published 9 October 2017 Low-index materials are key components in integrated Although naturally occurring materials with low refractive photonics and can enhance index contrast and improve per- indices are limited, there are significant research efforts to formance. Such materials can be constructed from porous artificially create low-index materials close to the index of materials, which generally lack mechanical strength and air. These new materials consist of porous structures through are difficult to integrate. Here we demonstrate enhanced to- various conventional fabrication techniques, such as oblique tal internal reflection (TIR) induced by integrating robust deposition [5–9], the sol-gel process [10], and chemical vapor nanolattice materials with periodic architectures between deposition [11]. Due to the air voids, the fabricated porous high-index media. The transmission measurement from structures can have effective refractive indices lower than the the multilayer stack illustrates a cutoff at about a 60° inci- solid material components. However, such materials typically dence angle, indicating an enhanced light trapping effect lack mechanical stability and can induce optical scattering be- through TIR. Light propagation in the nanolattice material cause of the random architectures of the structures. -
Why Doesn't the Sky Turn Green?
Why Doesn't The Sky Turn Green? Rushi Shah 09 November 2015 I was leafing through the US Army Corps of Engineers' Manual on Remote Sensing and learned about how Rayleigh scattering creates blue skies during the day and redish-orange sunsets. Well, everybody knows about blue skies and red sunsets, was the title just click-bait, you ask? No, but I'll get to that. 1 The Basics of Rayleigh Scattering Let's start with this whole Rayleigh scattering thing. Basically the idea is that particles in the atmosphere (like Oxygen and Nitrogen particles) scatter rays of light as they enter the atmosphere. How much the light is scattered is heavily dependent on the wavelength of the light. Remember how ROY-G-BIV represents the colors of the rainbow (and thus the spectrum of visible light)? Well that spectrum of light starts with long wavelength rays (reds, oranges, and yellows) and ends with short wavelength rays (blues, indigos, and violets). 1 The amount of light that is scattered (denoted with the variable I) is highly inversely proportional to the wavelength of the light (denoted with the greek letter ). Put simply, as the wavelength goes down, the light waves are scattered more quickly. 1 I / λ4 When sunlight hits the earth directly during mid-day, it travels through a small amount of atmosphere, and thus we see the wavelengths of light that are immediately scattered. Based on the equation above, the blues, indigos, and violets would be scattered first (and they blend together into the blue sky we see). As the sun sets, it goes through more and more particles to reach us. -
Light, Color, and Atmospheric Optics
Light, Color, and Atmospheric Optics GEOL 1350: Introduction To Meteorology 1 2 • During the scattering process, no energy is gained or lost, and therefore, no temperature changes occur. • Scattering depends on the size of objects, in particular on the ratio of object’s diameter vs wavelength: 1. Rayleigh scattering (D/ < 0.03) 2. Mie scattering (0.03 ≤ D/ < 32) 3. Geometric scattering (D/ ≥ 32) 3 4 • Gas scattering: redirection of radiation by a gas molecule without a net transfer of energy of the molecules • Rayleigh scattering: absorption extinction 4 coefficient s depends on 1/ . • Molecules scatter short (blue) wavelengths preferentially over long (red) wavelengths. • The longer pathway of light through the atmosphere the more shorter wavelengths are scattered. 5 • As sunlight enters the atmosphere, the shorter visible wavelengths of violet, blue and green are scattered more by atmospheric gases than are the longer wavelengths of yellow, orange, and especially red. • The scattered waves of violet, blue, and green strike the eye from all directions. • Because our eyes are more sensitive to blue light, these waves, viewed together, produce the sensation of blue coming from all around us. 6 • Rayleigh Scattering • The selective scattering of blue light by air molecules and very small particles can make distant mountains appear blue. The blue ridge mountains in Virginia. 7 • When small particles, such as fine dust and salt, become suspended in the atmosphere, the color of the sky begins to change from blue to milky white. • These particles are large enough to scatter all wavelengths of visible light fairly evenly in all directions. -
Project Horizon Report
Volume I · SUMMARY AND SUPPORTING CONSIDERATIONS UNITED STATES · ARMY CRD/I ( S) Proposal t c• Establish a Lunar Outpost (C) Chief of Ordnance ·cRD 20 Mar 1 95 9 1. (U) Reference letter to Chief of Ordnance from Chief of Research and Devel opment, subject as above. 2. (C) Subsequent t o approval by t he Chief of Staff of reference, repre sentatives of the Army Ballistic ~tissiles Agency indicat e d that supplementar y guidance would· be r equired concerning the scope of the preliminary investigation s pecified in the reference. In particular these r epresentatives requested guidance concerning the source of funds required to conduct the investigation. 3. (S) I envision expeditious development o! the proposal to establish a lunar outpost to be of critical innportance t o the p. S . Army of the future. This eva luation i s appar ently shar ed by the Chief of Staff in view of his expeditious a pproval and enthusiastic endorsement of initiation of the study. Therefore, the detail to be covered by the investigation and the subs equent plan should be as com plete a s is feas ible in the tin1e limits a llowed and within the funds currently a vailable within t he office of t he Chief of Ordnance. I n this time of limited budget , additional monies are unavailable. Current. programs have been scrutinized r igidly and identifiable "fat'' trimmed awa y. Thus high study costs are prohibitive at this time , 4. (C) I leave it to your discretion t o determine the source and the amount of money to be devoted to this purpose. -
ESSENTIALS of METEOROLOGY (7Th Ed.) GLOSSARY
ESSENTIALS OF METEOROLOGY (7th ed.) GLOSSARY Chapter 1 Aerosols Tiny suspended solid particles (dust, smoke, etc.) or liquid droplets that enter the atmosphere from either natural or human (anthropogenic) sources, such as the burning of fossil fuels. Sulfur-containing fossil fuels, such as coal, produce sulfate aerosols. Air density The ratio of the mass of a substance to the volume occupied by it. Air density is usually expressed as g/cm3 or kg/m3. Also See Density. Air pressure The pressure exerted by the mass of air above a given point, usually expressed in millibars (mb), inches of (atmospheric mercury (Hg) or in hectopascals (hPa). pressure) Atmosphere The envelope of gases that surround a planet and are held to it by the planet's gravitational attraction. The earth's atmosphere is mainly nitrogen and oxygen. Carbon dioxide (CO2) A colorless, odorless gas whose concentration is about 0.039 percent (390 ppm) in a volume of air near sea level. It is a selective absorber of infrared radiation and, consequently, it is important in the earth's atmospheric greenhouse effect. Solid CO2 is called dry ice. Climate The accumulation of daily and seasonal weather events over a long period of time. Front The transition zone between two distinct air masses. Hurricane A tropical cyclone having winds in excess of 64 knots (74 mi/hr). Ionosphere An electrified region of the upper atmosphere where fairly large concentrations of ions and free electrons exist. Lapse rate The rate at which an atmospheric variable (usually temperature) decreases with height. (See Environmental lapse rate.) Mesosphere The atmospheric layer between the stratosphere and the thermosphere. -
Atmospheric Optics Learning Module
Atmospheric Optics Learning Module Everything we see is the reflection of light and without light, everything would be dark. In this learning module, we will discuss the various wavelengths of light and how it is transmitted through Earth’s atmosphere to explain fascinating optical phenomena including why the sky is blue and how rainbows form! To get started, watch this video describing energy in the form of waves. A sundog and halo display in Greenland. Electromagnetic Spectrum (0 – 6:30) Source Electromagnetic Spectrum Electromagnetic (EM) radiation is light. Light you might see in a rainbow, or better yet, a double rainbow such as the one seen in Figure 1. But it is also radio waves, x-rays, and gamma rays. It is incredibly important because there are only two ways we can move energy from place to place. The first is using what is called a particle, or an object moving from place to place. The second way to move energy is through a wave. The interesting thing about EM radiation is that it is both a particle and a wave 1. Figure 1. Double rainbow Source 1 Created by Tyra Brown, Nicole Riemer, Eric Snodgrass and Anna Ortiz at the University of Illinois at Urbana- This work is licensed under a Creative Commons Attribution-ShareAlike 4.0 International License. Champaign. 2015-2016. Supported by the National Science Foundation CAREER Grant #1254428. There are many frequencies of EM radiation that we cannot see. So if we change the frequency, we might have radio waves, which we cannot see, but they are all around us! The same goes for x-rays you might get if you break a bone. -
Links to Education Standards • Introduction to Exoplanets • Glossary of Terms • Classroom Activities Table of Contents Photo © Michael Malyszko Photo © Michael
an Educator’s Guid E INSIDE • Links to Education Standards • Introduction to Exoplanets • Glossary of Terms • Classroom Activities Table of Contents Photo © Michael Malyszko Photo © Michael For The TeaCher About This Guide .............................................................................................................................................. 4 Connections to Education Standards ................................................................................................... 5 Show Synopsis .................................................................................................................................................. 6 Meet the “Stars” of the Show .................................................................................................................... 8 Introduction to Exoplanets: The Basics .................................................................................................. 9 Delving Deeper: Teaching with the Show ................................................................................................13 Glossary of Terms ..........................................................................................................................................24 Online Learning Tools ..................................................................................................................................26 Exoplanets in the News ..............................................................................................................................27 During -
Atmospheric Refraction
Z w o :::> l I ~ <x: '----1-'-1----- WAVE FR ON T Z = -rnX + Zal rn = tan e '----------------------X HORIZONTAL POSITION de = ~~ = (~~) tan8dz v FIG. 1. Origin of atmospheric refraction. DR. SIDNEY BERTRAM The Bunker-Ramo Corp. Canoga Park, Calif. Atmospheric Refraction INTRODUCTION it is believed that the treatment presented herein represents a new and interesting ap T IS WELL KNOWN that the geometry of I aerial photographs may be appreciably proach to the problem. distorted by refraction in the atmosphere at THEORETICAL DISCUSSION the time of exposure, and that to obtain maximum mapping accuracy it is necessary The origin of atmospheric refraction can to compensate this distortion as much as be seen by reference to Figure 1. A light ray knowledge permits. This paper presents a L is shown at an angle (J to the vertical in a solution of the refraction problem, including medium in which the velocity of light v varies a hand calculation for the ARDC Model 1 A. H. Faulds and Robert H. Brock, Jr., "Atmo Atmosphere, 1959. The effect of the curva spheric Refraction and its Distortion of Aerial ture of the earth on the refraction problem is Photography," PSOTOGRAMMETRIC ENGINEERING analyzed separately and shown to be negli Vol. XXX, No.2, March 1964. 2 H. H. Schmid, "A General Analytical Solution gible, except for rays approaching the hori to the Problem of Photogrammetry," Ballistic Re zontal. The problem of determining the re search Laboratories Report No. 1065, July 1959 fraction for a practical situation is also dis (ASTIA 230349). cussed. 3 D. C. -
The Min Min Light and the Fata Morgana Pettigrew
C L I N I C A L A N D E X P E R I M E N T A L OPTOMETRYThe Min Min light and the Fata Morgana Pettigrew COMMENTARY The Min Min light and the Fata Morgana An optical account of a mysterious Australian phenomenon Clin Exp Optom 2003; 86: 2: 109–120 John D Pettigrew BSc(Med) MSc MBBS Background: Despite intense interest in this mysterious Australian phenomenon, the FRS Min Min light has never been explained in a satisfactory way. Vision Touch and Hearing Research Methods and Results: An optical explanation of the Min Min light phenomenon is of- Centre, University of Queensland fered, based on a number of direct observations of the phenomenon, as well as a field demonstration, in the Channel Country of Western Queensland. This explanation is based on the inverted mirage or Fata Morgana, where light is refracted long distances over the horizon by the refractive index gradient that occurs in the layers of air during a temperature inversion. Both natural and man-made light sources can be involved, with the isolated light source making it difficult to recognise the features of the Fata Morgana that are obvious in daylight and with its unsuspected great distance contribut- ing to the mystery of its origins. Submitted: 11 September 2002 Conclusion: Many of the strange properties of the Min Min light are explicable in terms Revised: 2 December 2002 of the unusual optical conditions of the Fata Morgana, if account is also taken of the Accepted for publication: 3 December human factors that operate under these highly-reduced stimulus conditions involving a 2002 single isolated light source without reference landmarks. -
Atmospheric Optics
53 Atmospheric Optics Craig F. Bohren Pennsylvania State University, Department of Meteorology, University Park, Pennsylvania, USA Phone: (814) 466-6264; Fax: (814) 865-3663; e-mail: [email protected] Abstract Colors of the sky and colored displays in the sky are mostly a consequence of selective scattering by molecules or particles, absorption usually being irrelevant. Molecular scattering selective by wavelength – incident sunlight of some wavelengths being scattered more than others – but the same in any direction at all wavelengths gives rise to the blue of the sky and the red of sunsets and sunrises. Scattering by particles selective by direction – different in different directions at a given wavelength – gives rise to rainbows, coronas, iridescent clouds, the glory, sun dogs, halos, and other ice-crystal displays. The size distribution of these particles and their shapes determine what is observed, water droplets and ice crystals, for example, resulting in distinct displays. To understand the variation and color and brightness of the sky as well as the brightness of clouds requires coming to grips with multiple scattering: scatterers in an ensemble are illuminated by incident sunlight and by the scattered light from each other. The optical properties of an ensemble are not necessarily those of its individual members. Mirages are a consequence of the spatial variation of coherent scattering (refraction) by air molecules, whereas the green flash owes its existence to both coherent scattering by molecules and incoherent scattering