Mars Thermospheric Variability Revealed by MAVEN EUVM Solar Occultations: Structure at Aphelion and Perihelion, and Response to EUV Forcing E
Total Page:16
File Type:pdf, Size:1020Kb
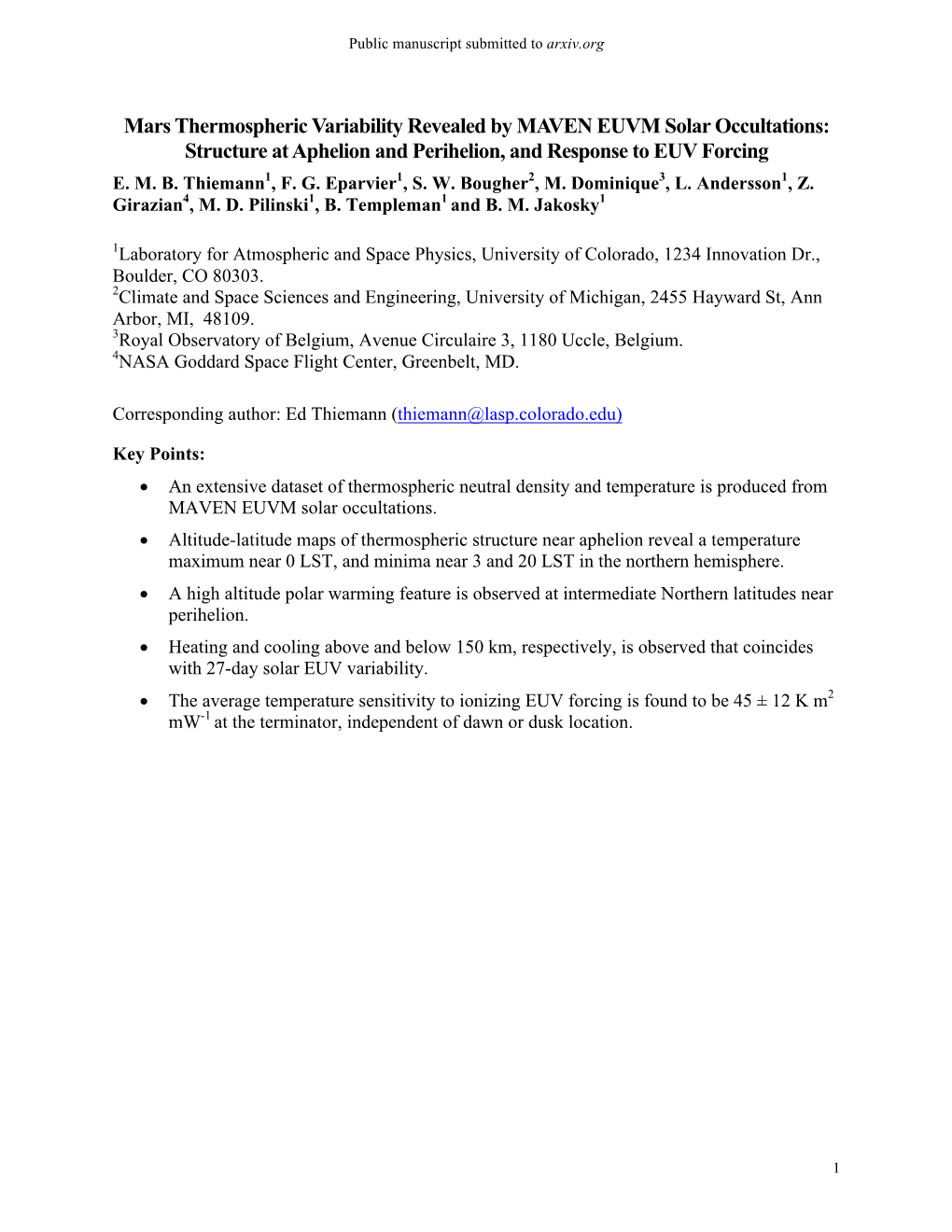
Load more
Recommended publications
-
Global Ionosphere-Thermosphere-Mesosphere (ITM) Mapping Across Temporal and Spatial Scales a White Paper for the NRC Decadal
Global Ionosphere-Thermosphere-Mesosphere (ITM) Mapping Across Temporal and Spatial Scales A White Paper for the NRC Decadal Survey of Solar and Space Physics Andrew Stephan, Scott Budzien, Ken Dymond, and Damien Chua NRL Space Science Division Overview In order to fulfill the pressing need for accurate near-Earth space weather forecasts, it is essential that future measurements include both temporal and spatial aspects of the evolution of the ionosphere and thermosphere. A combination of high altitude global images and low Earth orbit altitude profiles from simple, in-the-medium sensors is an optimal scenario for creating continuous, routine space weather maps for both scientific and operational interests. The method presented here adapts the vast knowledge gained using ultraviolet airglow into a suggestion for a next-generation, near-Earth space weather mapping network. Why the Ionosphere, Thermosphere, and Mesosphere? The ionosphere-thermosphere-mesosphere (ITM) region of the terrestrial atmosphere is a complex and dynamic environment influenced by solar radiation, energy transfer, winds, waves, tides, electric and magnetic fields, and plasma processes. Recent measurements showing how coupling to other regions also influences dynamics in the ITM [e.g. Immel et al., 2006; Luhr, et al, 2007; Hagan et al., 2007] has exposed the need for a full, three- dimensional characterization of this region. Yet the true level of complexity in the ITM system remains undiscovered primarily because the fundamental components of this region are undersampled on the temporal and spatial scales that are necessary to expose these details. The solar and space physics research community has been driven over the past decade toward answering scientific questions that have a high level of practical application and relevance. -
Marina Galand
Thermosphere - Ionosphere - Magnetosphere Coupling! Canada M. Galand (1), I.C.F. Müller-Wodarg (1), L. Moore (2), M. Mendillo (2), S. Miller (3) , L.C. Ray (1) (1) Department of Physics, Imperial College London, London, U.K. (2) Center for Space Physics, Boston University, Boston, MA, USA (3) Department of Physics and Astronomy, University College London, U.K. 1." Energy crisis at giant planets Credit: NASA/JPL/Space Science Institute 2." TIM coupling Cassini/ISS (false color) 3." Modeling of IT system 4." Comparison with observaons Cassini/UVIS 5." Outstanding quesAons (Pryor et al., 2011) SATURN JUPITER (Gladstone et al., 2007) Cassini/UVIS [UVIS team] Cassini/VIMS (IR) Credit: J. Clarke (BU), NASA [VIMS team/JPL, NASA, ESA] 1. SETTING THE SCENE: THE ENERGY CRISIS AT THE GIANT PLANETS THERMAL PROFILE Exosphere (EARTH) Texo 500 km Key transiLon region Thermosphere between the space environment and the lower atmosphere Ionosphere 85 km Mesosphere 50 km Stratosphere ~ 15 km Troposphere SOLAR ENERGY DEPOSITION IN THE UPPER ATMOSPHERE Solar photons ion, e- Neutral Suprathermal electrons B ion, e- Thermal e- Ionospheric Thermosphere Ne, Nion e- heang Te P, H * + Airglow Neutral atmospheric Exothermic reacAons heang IS THE SUN THE MAIN ENERGY SOURCE OF PLANERATY THERMOSPHERES? W Main energy source: UV solar radiaon Main energy source? EartH Outer planets CO2 atmospHeres Exospheric temperature (K) [aer Mendillo et al., 2002] ENERGY CRISIS AT THE GIANT PLANETS Observed values at low to mid-latudes solsce equinox Modeled values (Sun only) [Aer -
Soaring Weather
Chapter 16 SOARING WEATHER While horse racing may be the "Sport of Kings," of the craft depends on the weather and the skill soaring may be considered the "King of Sports." of the pilot. Forward thrust comes from gliding Soaring bears the relationship to flying that sailing downward relative to the air the same as thrust bears to power boating. Soaring has made notable is developed in a power-off glide by a conven contributions to meteorology. For example, soar tional aircraft. Therefore, to gain or maintain ing pilots have probed thunderstorms and moun altitude, the soaring pilot must rely on upward tain waves with findings that have made flying motion of the air. safer for all pilots. However, soaring is primarily To a sailplane pilot, "lift" means the rate of recreational. climb he can achieve in an up-current, while "sink" A sailplane must have auxiliary power to be denotes his rate of descent in a downdraft or in come airborne such as a winch, a ground tow, or neutral air. "Zero sink" means that upward cur a tow by a powered aircraft. Once the sailcraft is rents are just strong enough to enable him to hold airborne and the tow cable released, performance altitude but not to climb. Sailplanes are highly 171 r efficient machines; a sink rate of a mere 2 feet per second. There is no point in trying to soar until second provides an airspeed of about 40 knots, and weather conditions favor vertical speeds greater a sink rate of 6 feet per second gives an airspeed than the minimum sink rate of the aircraft. -
Sky Watch Heard Most Weekdays on WFWM, FSU's Public Supported
Night Highlights – Dec.2014 through Dec.2015 by Dr. Bob Doyle, Frostburg State Planetarium Dr. Doyle’s email is: [email protected]: His office phone number is (301) 687-7799 MOON – Earth’s companion both orbits Earth and rotates in 27.32 E. days so one side of moon always faces Earth (while other side is turned away from us). Moon’s cycle of lighted shapes (phases) lasts 29.53 E. days, as the phases also depend on direction of sun (appears to move 30 degrees eastward each month along zodiac). The moon is seen about 13 days growing in the evening from a slender crescent ( ) ) to Full, followed by an equal time shrinking (mainly seen in the a.m. sky) and then 3 days hidden in sun’s glare. Key Moon Phases (D) moon ½ full in evening (best for crater & mountain viewing ) & (O) full moon (see all lava plains) (Dec. ’14, 6 -O, 28 – D)) // (Jan. ’15, 4 - O, 26 - D), // (Feb. ’15, 3 – O, 25 - D) // (Mar. 5 – O, 27 – D) (Apr. 4 – O, 25 – D), // ( May 3 – O, 25 - D) // (Jun., 2- O, 27 – D)) // (Jul., 1 – O, 24 –D, 31 –O (Blue Moon)) (Aug., 22 – D, 29 – O) // (Sep., 20 – D, 27 – O (Harvest Moon)) // (Oct. 20 – D, 27 – O (Hunters’ Moon)) // (Nov., 19 – D, 25 – O) // (Dec., 18 – D, 25 – O (Long Night Moon)) (D = ½ full, O = full) THE 5 BRIGHT PLANETS (Mercury, Venus, Mars, Jupiter & Saturn): Uranus, Neptune are much dimmer. When high above horizon, planets appear as points of light that shine steadily. -
Thermosphere? Why Is It So Hot? Atmosphere Model and Thermosphere 5
UNIT 6, LESSON 1: EARTH LAYERS & ATMOSPHERE LET’S BUILD OUR EARTH AND THE ATMOSPHERE 1 & 2 Where is earth’s crust? What is the difference between the 1. Read/annotate the oceanic and continental crust? paragraph(s) Crust and Mantle Where is the earth’s mantle? What is a plate? What part of the 2. Answer the questions mantle is below the plates? that go with the 3 Where is the earth’s core? How is the core similar to the mantle? paragraph(s) Core 4 & 5 Where is the troposphere? What are you likely to find there? Name at least 3 things. 3. Show Mrs. Stoddard Troposphere and Stratosphere your answers Where is the stratosphere? Why would the air be warm in the 4. If you get it correct, get stratosphere? Where is the mesosphere? Why do meteors burn in this layer? a piece of your 6 & 7 Mesosphere Where is the thermosphere? Why is it so hot? atmosphere model And Thermosphere 5. Repeat until your 8 Where is the exosphere? Why do some experts ignore the exosphere and think the thermosphere is the outermost layer of Exosphere model is finished! the earth’s atmosphere? CHECK YOUR MODEL! Exosphere Thermosphere Mesosphere Stratosphere Troposphere Core Name Class Period Mantle Crust HOW DID WE DO ON THE ATMOSPHERE MODEL? LET’S SET UP OUR JOURNALS! Earth’s Layers and Atmosphere 1:301:291:281:271:261:251:241:231:221:211:201:191:181:171:161:151:141:131:121:111:101:091:081:071:061:051:041:031:021:011:000:590:580:570:560:550:540:530:520:510:500:490:480:470:460:450:440:430:420:410:400:390:380:370:360:350:340:330:320:310:300:290:280:270:260:250:240:230:220:210:200:190:180:170:160:150:140:130:120:110:100:090:080:070:060:050:040:030:020:01End -
The Blue Hours Dusk, Early September, Just Beneath the Arctic
The Blue Hours Dusk, early September, just beneath the Arctic Circle, by a tideline glacier in East Greenland. The cusp of the seasons, the cusp of the globe, the cusp of the land, and the day’s cusp too: twilight, the blue hours. At this latitude, at this time of year, dusk lasts for two or three hours. We have returned from a long mountain day: pitched climbing up steep slabs and over snow slopes to a towered summit, from which height we could see the great inland ice-cap itself. Then down, late in the day, the darkness thickening around us, and the sun dropping fast behind the western peaks. So we sit together back at camp as the last light gathers on the water of the fjord, on icebergs, on the quartz seams in the white boulder-field above our tents. Twilight specifies the landscape in this way – but it also disperses it. Relations between objects are loosened, such that shape-shifts occur. Just before full night falls, and the aurora borealis begins, a powerful hallucination occurs. My tired eyes start to see every pale stone around our tent not as boulder but as bear, polar bear, pure bear, crouched for the spring. Across the Northern hemisphere, twilight is known as the trickster-time: breeder of delusion, feeder of fantasies, zone of becomings. In Greek, dusk is called lykophos, ‘wolf-light’. In Austria, too, it is Wolflicht. In French it is the phase entre chien et loup, ‘between dog and wolf’: the time when, as Chrystel Lebas has written, ‘it is nearly impossible to tell the difference between the howling sound coming from the two animals, when the domestic and familiar transform into the wild.’ I do not know the Greenlandic word for dusk, but perhaps it would translate as ‘bear-light’. -
Astrobiology in Low Earth Orbit
The O/OREOS Mission – Astrobiology in Low Earth Orbit P. Ehrenfreund1, A.J. Ricco2, D. Squires2, C. Kitts3, E. Agasid2, N. Bramall2, K. Bryson4, J. Chittenden2, C. Conley5, A. Cook2, R. Mancinelli4, A. Mattioda2, W. Nicholson6, R. Quinn7, O. Santos2, G. Tahu5, M. Voytek5, C. Beasley2, L. Bica3, M. Diaz-Aguado2, C. Friedericks2, M. Henschke2, J.W. Hines2, D. Landis8, E. Luzzi2, D. Ly2, N. Mai2, G. Minelli2, M. McIntyre2, M. Neumann3, M. Parra2, M. Piccini2, R. Rasay3, R. Ricks2, A. Schooley2, E. Stackpole2, L. Timucin2, B. Yost2, A. Young3 1Space Policy Institute, Washington, DC, USA [email protected], 2NASA Ames Research Center, Moffett Field, CA, USA, 3Robotic Systems Laboratory, Santa Clara University, Santa Clara, CA, USA, 4Bay Area Environmental Research Institute, Sonoma, CA, USA, 5NASA Headquarters, Washington DC, USA, 6University of Florida, Gainesville, FL, USA, 7SETI Institute, Mountain View, CA, USA, 8Draper Laboratory, Cambridge, MA, USA Abstract. The O/OREOS (Organism/Organic Exposure to Orbital Stresses) nanosatellite is the first science demonstration spacecraft and flight mission of the NASA Astrobiology Small- Payloads Program (ASP). O/OREOS was launched successfully on November 19, 2010, to a high-inclination (72°), 650-km Earth orbit aboard a US Air Force Minotaur IV rocket from Kodiak, Alaska. O/OREOS consists of 3 conjoined cubesat (each 1000 cm3) modules: (i) a control bus, (ii) the Space Environment Survivability of Living Organisms (SESLO) experiment, and (iii) the Space Environment Viability of Organics (SEVO) experiment. Among the innovative aspects of the O/OREOS mission are a real-time analysis of the photostability of organics and biomarkers and the collection of data on the survival and metabolic activity for micro-organisms at 3 times during the 6-month mission. -
The O/OREOS Mission—Astrobiology in Low Earth Orbit
Acta Astronautica 93 (2014) 501–508 Contents lists available at ScienceDirect Acta Astronautica journal homepage: www.elsevier.com/locate/actaastro The O/OREOS mission—Astrobiology in low Earth orbit P. Ehrenfreund a,n, A.J. Ricco b, D. Squires b, C. Kitts c, E. Agasid b, N. Bramall b, K. Bryson d, J. Chittenden b, C. Conley e, A. Cook b, R. Mancinelli d, A. Mattioda b, W. Nicholson f, R. Quinn g, O. Santos b,G.Tahue,M.Voyteke, C. Beasley b,L.Bicac, M. Diaz-Aguado b, C. Friedericks b,M.Henschkeb,D.Landish, E. Luzzi b,D.Lyb, N. Mai b, G. Minelli b,M.McIntyreb,M.Neumannc, M. Parra b, M. Piccini b, R. Rasay c,R.Ricksb, A. Schooley b, E. Stackpole b, L. Timucin b,B.Yostb, A. Young c a Space Policy Institute, Washington DC, USA b NASA Ames Research Center, Moffett Field, CA, USA c Robotic Systems Laboratory, Santa Clara University, Santa Clara, CA, USA d Bay Area Environmental Research Institute, Sonoma, CA, USA e NASA Headquarters, Washington DC, USA f University of Florida, Gainesville, FL, USA g SETI Institute, Mountain View, CA, USA h Draper Laboratory, Cambridge, MA, USA article info abstract Article history: The O/OREOS (Organism/Organic Exposure to Orbital Stresses) nanosatellite is the first Received 19 December 2011 science demonstration spacecraft and flight mission of the NASA Astrobiology Small- Received in revised form Payloads Program (ASP). O/OREOS was launched successfully on November 19, 2010, to 22 June 2012 a high-inclination (721), 650-km Earth orbit aboard a US Air Force Minotaur IV rocket Accepted 18 September 2012 from Kodiak, Alaska. -
Diffuse Electron Precipitation in Magnetosphere-Ionosphere- Thermosphere Coupling
EGU21-6342 https://doi.org/10.5194/egusphere-egu21-6342 EGU General Assembly 2021 © Author(s) 2021. This work is distributed under the Creative Commons Attribution 4.0 License. Diffuse electron precipitation in magnetosphere-ionosphere- thermosphere coupling Dong Lin1, Wenbin Wang1, Viacheslav Merkin2, Kevin Pham1, Shanshan Bao3, Kareem Sorathia2, Frank Toffoletto3, Xueling Shi1,4, Oppenheim Meers5, George Khazanov6, Adam Michael2, John Lyon7, Jeffrey Garretson2, and Brian Anderson2 1High Altitude Observatory, National Center for Atmospheric Research, Boulder CO, United States of America 2Applied Physics Laboratory, Johns Hopkins University, Laurel MD, USA 3Department of Physics and Astronomy, Rice University, Houston TX, USA 4Bradley Department of Electrical and Computer Engineering, Virginia Tech, Blacksburg VA, USA 5Astronomy Department, Boston University, Boston MA, USA 6Goddard Space Flight Center, NASA, Greenbelt MD, USA 7Department of Physics and Astronomy, Dartmouth College, Hanover NH, USA Auroral precipitation plays an important role in magnetosphere-ionosphere-thermosphere (MIT) coupling by enhancing ionospheric ionization and conductivity at high latitudes. Diffuse electron precipitation refers to scattered electrons from the plasma sheet that are lost in the ionosphere. Diffuse precipitation makes the largest contribution to the total precipitation energy flux and is expected to have substantial impacts on the ionospheric conductance and affect the electrodynamic coupling between the magnetosphere and ionosphere-thermosphere. -
Midnight Sun, Part II by PA Lassiter
Midnight Sun, Part II by PA Lassiter . N.B. These chapters are based on characters created by Stephenie Meyer in Twilight, the novel. The title used here, Midnight Sun, some of the chapter titles, and all the non-interior dialogue between Edward and Bella are copyright Stephenie Meyer. The first half of Ms. Meyer’s rough-draft novel, of which this is a continuation, can be found at her website here: http://www.stepheniemeyer.com/pdf/midnightsun_partial_draft4.pdf 12. COMPLICATIONSPart B It was well after midnight when I found myself slipping through Bella’s window. This was becoming a habit that, in the light of day, I knew I should attempt to curb. But after nighttime fell and I had huntedfor though these visits might be irresponsible, I was determined they not be recklessall of my resolve quickly faded. There she lay, the sheet and blanket coiled around her restless body, her feet bound up outside the covers. I inhaled deeply through my nose, welcoming the searing pain that coursed down my throat. As always, Bella’s bedroom was warm and humid and saturated with her scent. Venom flowed into my mouth and my muscles tensed in readiness. But for what? Could I ever train my body to give up this devilish reaction to my beloved’s smell? I feared not. Cautiously, I held my breath and moved to her bedside. I untangled the bedclothes and spread them carefully over her again. She twitched suddenly, her legs scissoring as she rolled to her other side. I froze. “Edward,” she breathed. -
Excerpts from Dusk of Dawn: an Essay Toward an Autobiography Of
DUSK OF DAWN An Essay Toward an Autobiography of a Race Concept W. E. B. Du Bois Series Editor, Henry Louis Gates, Jr. Introduction by K. Anthony Appiah OXFORD UNIVERSITY PRESS Contents SERIES INTRODUCTION: THE BLACK LETTERS ON THE SIGN xi INTRODUCTION xxv APOLOGY xxxiii I. THEPLOT 1 II. A NEW ENGLAND BOY AND RECONSTRUCTION 4 III. EDUCATION IN THE LAST DECADES OF THE NINETEENTH CENTURY 13 IV. SCIENCE AND EMPIRE 26 V. THE CONCEPT OF RACE 49 VI. THE WHITE WORLD 68 VII. THE COLORED WORLD WITHIN 88 VIII. PROPAGANDA AND WORLD WAR 111 IX. REVOLUTION 134 INDEX 163 WILLIAM EDWARD BURGHARDT DUBOIS: A CHRONOLOGY 171 SELECTED BIBLIOGRAPHY 179 ix CHAPTER VII The Colored World Within Not only do white men but also colored men forget the facts of the Negro's dou ble environment. The Negro American has for his environment not only the white surrounding world, but also, and touching him usually much more nearly and compellingly, is the environment furnished by his own colored group. There are exceptions, of course, but this is the rule. The American Negro, therefore, is surrounded and conditioned by the concept which he has of white people and he is treated in accordance with the concept they have of him. On the other hand, so far as his own people are concerned, he is in direct contact with individuals and facts. He fits into this environment more or less willingly. It gives him a social world and mental peace. On the other hand and especially if in education and ambition and income he is above the average culture of his group, he is often resentful of its environilcg power; partly because he does not recognize its power and partly because he is determined to consider himself part of the white group from which, in fact, he is excluded. -
George Orwell in His Centenary Year a Catalan Perspective
THE ANNUAL JOAN GILI MEMORIAL LECTURE MIQUEL BERGA George Orwell in his Centenary Year A Catalan Perspective THE ANGLO-CATALAN SOCIETY 2003 © Miquel Berga i Bagué This edition: The Anglo-Catalan Society Produced and typeset by Hallamshire Publications Ltd, Porthmadog. This is the fifth in the regular series of lectures convened by The Anglo-Catalan Society, to be delivered at its annual conference, in commemoration of the figure of Joan Lluís Gili i Serra (1907-1998), founder member of the Society and Honorary Life President from 1979. The object of publication is to ensure wider diffusion, in English, for an address to the Society given by a distinguished figure of Catalan letters whose specialism coincides with an aspect of the multiple interests and achievements of Joan Gili, as scholar, bibliophile and translator. This lecture was given by Miquel Berga at Aberdare Hall, University of Wales (Cardiff), on 16 November 2002. Translation of the text of the lecture and general editing of the publication were the responsibility of Alan Yates, with the cooperation of Louise Johnson. We are grateful to Miquel Berga himself and to Iolanda Pelegrí of the Institució de les Lletres Catalanes for prompt and sympathetic collaboration. Thanks are also due to Pauline Climpson and Jenny Sayles for effective guidance throughout the editing and production stages. Grateful acknowledgement is made of regular sponsorship of The Annual Joan Gili Memorial Lectures provided by the Institució de les Lletres Catalanes, and of the grant towards publication costs received from the Fundació Congrés de Cultura Catalana. The author Miquel Berga was born in Salt (Girona) in 1952.