1 Aberrant Astrocyte Protein Secretion Contributes to Altered
Total Page:16
File Type:pdf, Size:1020Kb
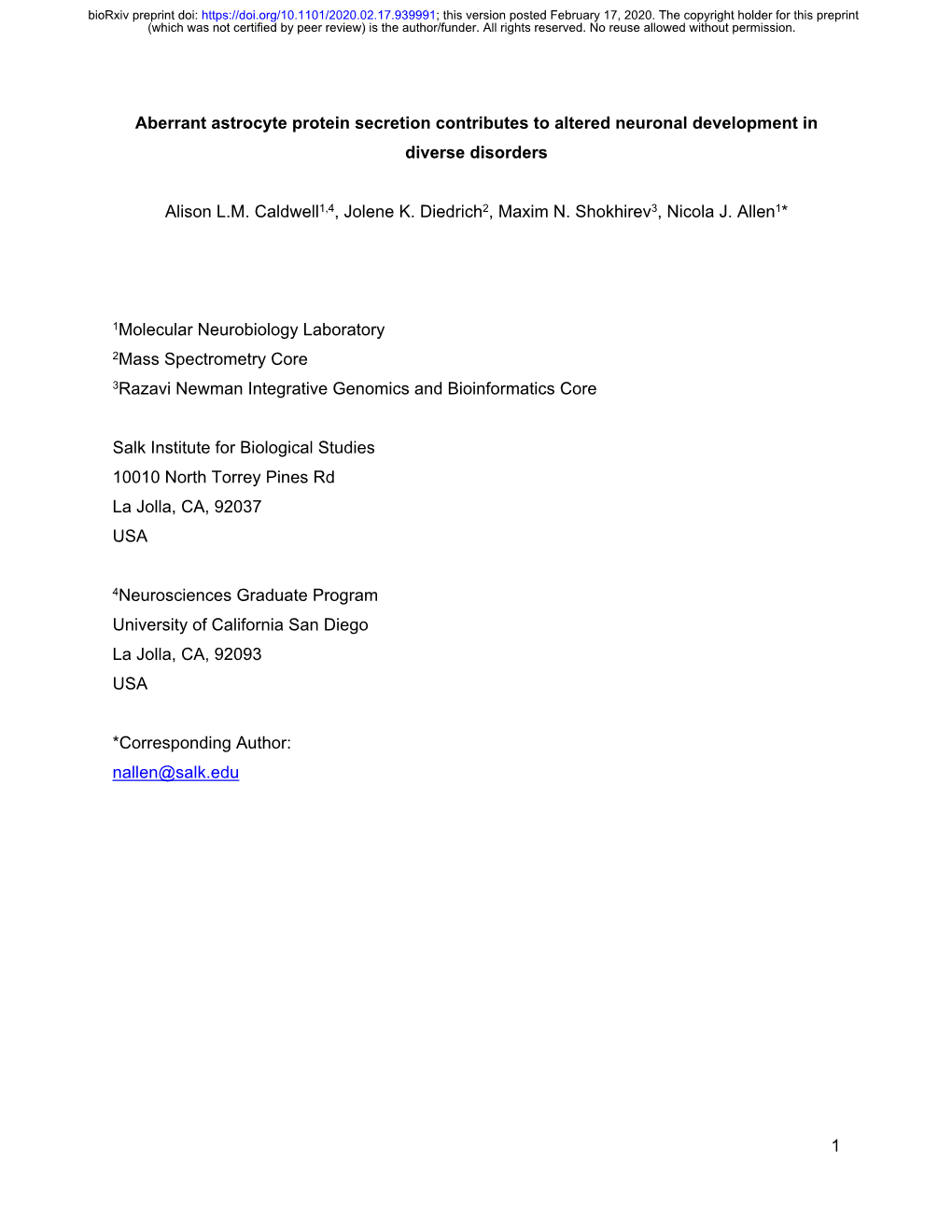
Load more
Recommended publications
-
RNA-Binding Protein Network Alteration Causes Aberrant Axon
bioRxiv preprint doi: https://doi.org/10.1101/2020.08.26.268631; this version posted August 26, 2020. The copyright holder for this preprint (which was not certified by peer review) is the author/funder, who has granted bioRxiv a license to display the preprint in perpetuity. It is made available under aCC-BY-NC-ND 4.0 International license. 1 RNA-binding protein network alteration causes aberrant axon 2 branching and growth phenotypes in FUS ALS mutant motoneurons 3 4 Maria Giovanna Garone1, Nicol Birsa2,3, Maria Rosito4, Federico Salaris1,4, Michela Mochi1, Valeria 5 de Turris4, Remya R. Nair5, Thomas J. Cunningham5, Elizabeth M. C. Fisher2, Pietro Fratta2 and 6 Alessandro Rosa1,4,6,* 7 8 1. Department of Biology and Biotechnology Charles Darwin, Sapienza University of Rome, P.le 9 A. Moro 5, 00185 Rome, Italy 10 2. UCL Queen Square Institute of Neurology, University College London, London, WC1N 3BG, 11 UK 12 3. UK Dementia Research Institute, University College London, London, WC1E 6BT, UK 13 4. Center for Life Nano Science, Istituto Italiano di Tecnologia, Viale Regina Elena 291, 00161 14 Rome, Italy 15 5. MRC Harwell Institute, Oxfordshire, OX11 0RD, UK 16 6. Laboratory Affiliated to Istituto Pasteur Italia-Fondazione Cenci Bolognetti, Department of 17 Biology and Biotechnology Charles Darwin, Sapienza University of Rome, Viale Regina Elena 18 291, 00161 Rome, Italy 19 20 * Corresponding author: [email protected]; Tel: +39-0649255218 1 bioRxiv preprint doi: https://doi.org/10.1101/2020.08.26.268631; this version posted August 26, 2020. The copyright holder for this preprint (which was not certified by peer review) is the author/funder, who has granted bioRxiv a license to display the preprint in perpetuity. -
Analysis of Proteins That Rapidly Change Upon Mechanistic
crossmark Research © 2016 by The American Society for Biochemistry and Molecular Biology, Inc. This paper is available on line at http://www.mcponline.org Analysis of Proteins That Rapidly Change Upon Mechanistic/Mammalian Target of Rapamycin Complex 1 (mTORC1) Repression Identifies Parkinson Protein 7 (PARK7) as a Novel Protein Aberrantly Expressed in Tuberous Sclerosis Complex (TSC)*□S Farr Niere‡§¶ʈ§§, Sanjeev Namjoshi‡§ §§, Ehwang Song**, Geoffrey A. Dilly‡§¶, Grant Schoenhard‡‡, Boris V. Zemelman‡§¶, Yehia Mechref**, and Kimberly F. Raab-Graham‡§¶ʈ‡‡¶¶ Many biological processes involve the mechanistic/mam- ally alters the expression of proteins associated with malian target of rapamycin complex 1 (mTORC1). Thus, epilepsy, Alzheimer’s disease, and autism spectrum the challenge of deciphering mTORC1-mediated func- disorder—neurological disorders that exhibit elevated tions during normal and pathological states in the central mTORC1 activity. Through a protein–protein interaction nervous system is challenging. Because mTORC1 is at the network analysis, we have identified common proteins core of translation, we have investigated mTORC1 func- shared among these mTORC1-related diseases. One such tion in global and regional protein expression. Activation protein is Parkinson protein 7, which has been implicated of mTORC1 has been generally regarded to promote in Parkinson’s disease, yet not associated with epilepsy, translation. Few but recent works have shown that sup- Alzheimers disease, or autism spectrum disorder. To ver- pression of mTORC1 can also promote local protein syn- ify our finding, we provide evidence that the protein ex- thesis. Moreover, excessive mTORC1 activation during pression of Parkinson protein 7, including new protein diseased states represses basal and activity-induced pro- synthesis, is sensitive to mTORC1 inhibition. -
A Computational Approach for Defining a Signature of Β-Cell Golgi Stress in Diabetes Mellitus
Page 1 of 781 Diabetes A Computational Approach for Defining a Signature of β-Cell Golgi Stress in Diabetes Mellitus Robert N. Bone1,6,7, Olufunmilola Oyebamiji2, Sayali Talware2, Sharmila Selvaraj2, Preethi Krishnan3,6, Farooq Syed1,6,7, Huanmei Wu2, Carmella Evans-Molina 1,3,4,5,6,7,8* Departments of 1Pediatrics, 3Medicine, 4Anatomy, Cell Biology & Physiology, 5Biochemistry & Molecular Biology, the 6Center for Diabetes & Metabolic Diseases, and the 7Herman B. Wells Center for Pediatric Research, Indiana University School of Medicine, Indianapolis, IN 46202; 2Department of BioHealth Informatics, Indiana University-Purdue University Indianapolis, Indianapolis, IN, 46202; 8Roudebush VA Medical Center, Indianapolis, IN 46202. *Corresponding Author(s): Carmella Evans-Molina, MD, PhD ([email protected]) Indiana University School of Medicine, 635 Barnhill Drive, MS 2031A, Indianapolis, IN 46202, Telephone: (317) 274-4145, Fax (317) 274-4107 Running Title: Golgi Stress Response in Diabetes Word Count: 4358 Number of Figures: 6 Keywords: Golgi apparatus stress, Islets, β cell, Type 1 diabetes, Type 2 diabetes 1 Diabetes Publish Ahead of Print, published online August 20, 2020 Diabetes Page 2 of 781 ABSTRACT The Golgi apparatus (GA) is an important site of insulin processing and granule maturation, but whether GA organelle dysfunction and GA stress are present in the diabetic β-cell has not been tested. We utilized an informatics-based approach to develop a transcriptional signature of β-cell GA stress using existing RNA sequencing and microarray datasets generated using human islets from donors with diabetes and islets where type 1(T1D) and type 2 diabetes (T2D) had been modeled ex vivo. To narrow our results to GA-specific genes, we applied a filter set of 1,030 genes accepted as GA associated. -
Agrin Differentially Regulates the Rates of Axonal and Dendritic Elongation in Cultured Hippocampal Neurons
The Journal of Neuroscience, September 1, 2001, 21(17):6802–6809 Agrin Differentially Regulates the Rates of Axonal and Dendritic Elongation in Cultured Hippocampal Neurons Kristina B. Mantych and Adriana Ferreira Institute for Neuroscience and Department of Cell and Molecular Biology, Northwestern University Medical School, Chicago, Illinois 60611 In the present study, we examined the role of agrin in axonal elongation and branching were paralleled by changes in the and dendritic elongation in central neurons. Dissociated hip- composition of the cytoskeleton. In the presence of agrin, there pocampal neurons were grown in the presence of either recom- was an upregulation of the expression of microtubule- binant agrin or antisense oligonucleotides designed to block associated proteins MAP1B, MAP2, and tau. In contrast, a agrin expression. Our results indicate that agrin differentially downregulation of the expression of these MAPs was detected regulates axonal and dendritic growth. Recombinant agrin de- in agrin-depleted cells. Taken collectively, these results suggest creased the rate of elongation of main axons but induced the an important role for agrin as a trigger of the transcription of formation of axonal branches. On the other hand, agrin induced neuro-specific genes involved in neurite elongation and branch- both dendritic elongation and dendritic branching. Conversely, ing in central neurons. cultured hippocampal neurons depleted of agrin extended longer, nonbranched axons and shorter dendrites when com- Key words: agrin; neurite outgrowth; microtubule-associated pared with controls. These changes in the rates of neurite proteins; CREB; antisense oligonucleotides; axons and dendrites Agrin, an extracellular matrix protein, is synthesized by motor Conversely, neurons depleted of agrin elongated longer axons neurons and transported to their terminals where it is released when compared with control ones (Ferreira 1999; Serpinskaya et (Magill-Solc and McMahan, 1988; Martinou et al., 1991; Ruegg et al., 1999). -
DNA Methylation, Mechanisms of FMR1 Inactivation and Therapeutic Perspectives for Fragile X Syndrome
biomolecules Review DNA Methylation, Mechanisms of FMR1 Inactivation and Therapeutic Perspectives for Fragile X Syndrome Veronica Nobile 1, Cecilia Pucci 1, Pietro Chiurazzi 1,2 , Giovanni Neri 1,3 and Elisabetta Tabolacci 1,* 1 Sezione di Medicina Genomica, Dipartimento Scienze della Vita e Sanità Pubblica, Fondazione Policlinico Universitario A. Gemelli IRCCS, Università Cattolica del Sacro Cuore, 00168 Rome, Italy; [email protected] (V.N.); [email protected] (C.P.); [email protected] (P.C.); [email protected] (G.N.) 2 Fondazione Policlinico Universitario A. Gemelli IRCCS, UOC Genetica Medica, 00168 Rome, Italy 3 Greenwood Genetic Center, JC Self Research Institute, Greenwood, SC 29646, USA * Correspondence: [email protected]; Tel.: +39-06-30154606 Abstract: Among the inherited causes of intellectual disability and autism, Fragile X syndrome (FXS) is the most frequent form, for which there is currently no cure. In most FXS patients, the FMR1 gene is epigenetically inactivated following the expansion over 200 triplets of a CGG repeat (FM: full mutation). FMR1 encodes the Fragile X Mental Retardation Protein (FMRP), which binds several mRNAs, mainly in the brain. When the FM becomes methylated at 10–12 weeks of gestation, the FMR1 gene is transcriptionally silent. The molecular mechanisms involved in the epigenetic silencing are not fully elucidated. Among FXS families, there is a rare occurrence of males carrying a FM, which remains active because it is not methylated, thus ensuring enough FMRPs to allow for an intellectual development within normal range. Which mechanisms are responsible for sparing these individuals from being affected by FXS? In order to answer this critical question, which may have possible implications for FXS therapy, several potential epigenetic mechanisms have been described. -
Therapeutic Strategies in Fragile X Syndrome: Dysregulated Mglur Signaling and Beyond
Neuropsychopharmacology REVIEWS (2012) 37, 178–195 & 2012 American College of Neuropsychopharmacology All rights reserved 0893-133X/12 ............................................................................................................................................................... REVIEW 178 www.neuropsychopharmacology.org Therapeutic Strategies in Fragile X Syndrome: Dysregulated mGluR Signaling and Beyond 1 ,2 ,1,3 Christina Gross , Elizabeth M Berry-Kravis* and Gary J Bassell* 1 2 Department of Cell Biology, Emory University School of Medicine, Atlanta, GA, USA; Departments of Pediatrics, Neurology, 3 and Biochemistry, Rush University Medical Center, Chicago, IL, USA; Department of Neurology, Emory University School of Medicine, Atlanta, GA, USA Fragile X syndrome (FXS) is an inherited neurodevelopmental disease caused by loss of function of the fragile X mental retardation protein (FMRP). In the absence of FMRP, signaling through group 1 metabotropic glutamate receptors is elevated and insensitive to stimulation, which may underlie many of the neurological and neuropsychiatric features of FXS. Treatment of FXS animal models with negative allosteric modulators of these receptors and preliminary clinical trials in human patients support the hypothesis that metabotropic glutamate receptor signaling is a valuable therapeutic target in FXS. However, recent research has also shown that FMRP may regulate diverse aspects of neuronal signaling downstream of several cell surface receptors, suggesting a possible new route to more direct disease-targeted therapies. Here, we summarize promising recent advances in basic research identifying and testing novel therapeutic strategies in FXS models, and evaluate their potential therapeutic benefits. We provide an overview of recent and ongoing clinical trials motivated by some of these findings, and discuss the challenges for both basic science and clinical applications in the continued development of effective disease mechanism-targeted therapies for FXS. -
Localization and Alternative Splicing of Agrin
The Journal of Neuroscience, March 1994, 14(3): 1141-l 152 Localization and Alternative Splicing of Agrin mRNA in Adult Rat Brain: Transcripts Encoding lsoforms that Aggregate Acetylcholine Receptors Are Not Restricted to Cholinergic Regions Lawrence T. O’Connor, Julie C. Lauterborn, Christine M. Gall, and Martin A. Smith Department of Anatomy and Neurobiology, University of California at Irvine, Irvine, California 92717 Agrin is a protein implicated in the formation and mainte- that direct their organization. In comparison, much more is nance of the neuromuscular junction. In addition to motor known about the neuromuscularjunction. Like other chemical neurons, agrin mRNA has been detected in the brains of synapses,the neuromuscularjunction is characterized by spe- embryonic rat and chick and adult marine ray, suggesting cializations that adapt it for its role in synaptic transmission. that this molecule may also be involved in the formation of In particular, the postsynaptic apparatus is associatedwith a synapses between neurons. As a step toward understanding high concentration of acetylcholine receptors (AChR). The ac- agrin’s role in the CNS, we utilized Northern blot and in situ cumulation of AChR is an early event in development of the hybridization techniques to analyze the regional distribution neuromuscularjunction and has been shown to be the result of and cellular localization of agrin mRNA in the spinal cord inductive interactions between motor neuronsand musclefibers and brain of adult rats. The results of these studies indicate they innervate (reviewed in Hall and Sanes, 1993). Current ev- that the agrin mRNA is expressed predominantly by neurons idence suggeststhat agrin, a synaptic basal lamina protein, me- broadly distributed throughout the adult CNS. -
Role of Phosphodiesterases in the Pathophysiology of Neurodevelopmental Disorders
Molecular Psychiatry https://doi.org/10.1038/s41380-020-00997-9 REVIEW ARTICLE Role of phosphodiesterases in the pathophysiology of neurodevelopmental disorders 1 2 Sébastien Delhaye ● Barbara Bardoni Received: 4 July 2020 / Revised: 3 December 2020 / Accepted: 9 December 2020 © The Author(s), under exclusive licence to Springer Nature Limited part of Springer Nature 2021. This article is published with open access Abstract Phosphodiesterases (PDEs) are enzymes involved in the homeostasis of both cAMP and cGMP. They are members of a family of proteins that includes 11 subfamilies with different substrate specificities. Their main function is to catalyze the hydrolysis of cAMP, cGMP, or both. cAMP and cGMP are two key second messengers that modulate a wide array of intracellular processes and neurobehavioral functions, including memory and cognition. Even if these enzymes are present in all tissues, we focused on those PDEs that are expressed in the brain. We took into consideration genetic variants in patients affected by neurodevelopmental disorders, phenotypes of animal models, and pharmacological effects of PDE inhibitors, a class of drugs in rapid evolution and increasing application to brain disorders. Collectively, these data indicate the potential 1234567890();,: 1234567890();,: of PDE modulators to treat neurodevelopmental diseases characterized by learning and memory impairment, alteration of behaviors associated with depression, and deficits in social interaction. Indeed, clinical trials are in progress to treat patients with Alzheimer’s disease, schizophrenia, depression, and autism spectrum disorders. Among the most recent results, the application of some PDE inhibitors (PDE2A, PDE3, PDE4/4D, and PDE10A) to treat neurodevelopmental diseases, including autism spectrum disorders and intellectual disability, is a significant advance, since no specific therapies are available for these disorders that have a large prevalence. -
Altered Gut Microbiota in a Fragile X Syndrome Mouse Model
fnins-15-653120 May 26, 2021 Time: 10:27 # 1 ORIGINAL RESEARCH published: 26 May 2021 doi: 10.3389/fnins.2021.653120 Altered Gut Microbiota in a Fragile X Syndrome Mouse Model Francisco Altimiras1,2*, José Antonio Garcia1, Ismael Palacios-García3,4, Michael J. Hurley5, Robert Deacon6,7, Bernardo González8,9 and Patricia Cogram6,7* 1 Faculty of Engineering, Pontificia Universidad Católica de Valparaíso, Valparaíso, Chile, 2 Faculty of Engineering and Business, Universidad de las Américas, Santiago, Chile, 3 School of Psychology, Pontificia Universidad Católica de Chile, Santiago, Chile, 4 Centro de Estudios en Neurociencia Humana y Neuropsicología, Facultad de Psicología, Universidad Diego Portales, Santiago, Chile, 5 Biological Sciences, Faculty of Environmental and Life Sciences, University of Southampton, Southampton, United Kingdom, 6 Department of Genetics, Institute of Ecology and Biodiversity (IEB), Faculty of Sciences, Universidad de Chile, Santiago, Chile, 7 FRAXA-DVI, FRAXA Research Foundation, Santiago, Chile, 8 Faculty of Engineering and Sciences, Universidad Adolfo Ibáñez, Santiago, Chile, 9 Center of Applied Ecology and Sustainability (CAPES), Santiago, Chile The human gut microbiome is the ecosystem of microorganisms that live in the human digestive system. Several studies have related gut microbiome variants to metabolic, immune and nervous system disorders. Fragile X syndrome (FXS) is a neurodevelopmental disorder considered the most common cause of inherited intellectual disability and the leading monogenetic cause of autism. -
Impaired Presynaptic Long-Term Potentiation in the Anterior Cingulate Cortex of Fmr1 Knock-Out Mice
The Journal of Neuroscience, February 4, 2015 • 35(5):2033–2043 • 2033 Cellular/Molecular Impaired Presynaptic Long-Term Potentiation in the Anterior Cingulate Cortex of Fmr1 Knock-out Mice Kohei Koga,1,2* Ming-Gang Liu,1,3* Shuang Qiu,1,2* Qian Song,1,2 Gerile O’Den,2 Tao Chen,1,2,4 and Min Zhuo1,2 1Department of Physiology, Faculty of Medicine, University of Toronto, Medical Science Building, Toronto, Ontario, M5S 1A8, Canada, 2Center for Neuron and Disease, Frontier Institutes of Science and Technology, Xi’an Jiaotong University, Xi’an, Shanxi 710049, China, 3Department of Anatomy and Histology and Embryology, Institute of Medical Sciences, Shanghai Jiao Tong University School of Medicine, Shanghai 200025, China, and 4Department of Anatomy and KK Leung Brain Research Center, Fourth Military Medical University, Xi’an, Shanxi 710032, China Fragile X syndrome is a common inherited form of mental impairment. Fragile X mental retardation protein (FMRP) plays important roles in the regulation of synaptic protein synthesis, and loss of FMRP leads to deficits in learning-related synaptic plasticity and behavioraldisability.Previousstudiesmostlyfocusonpostsynapticlong-termpotentiation(LTP)inFmr1knock-out(KO)mice.Here,we investigatetheroleofFMRPinpresynapticLTP(pre-LTP)intheadultmouseanteriorcingulatecortex(ACC).Low-frequencystimulation induced LTP in layer II/III pyramidal neurons under the voltage-clamp mode. Paired-pulse ratio, which is a parameter for presynaptic changes, was decreased after the low-frequency stimulation in Fmr1 wild-type (WT) mice. Cingulate pre-LTP was abolished in Fmr1 KO mice. We also used a 64-electrode array system for field EPSP recording and found that the combination of low-frequency stimulation paired with a GluK1-containing kainate receptor agonist induced NMDA receptor-independent and metabotropic glutamate receptor- dependentpre-LTPintheWTmice.ThispotentiationwasblockedinFmr1KOmice.BiochemicalexperimentsshowedthatFmr1KOmice displayed altered translocation of protein kinase A subunits in the ACC. -
Genomics of Mature and Immature Olfactory Sensory Neurons Melissa D
University of Kentucky UKnowledge Physiology Faculty Publications Physiology 8-15-2012 Genomics of Mature and Immature Olfactory Sensory Neurons Melissa D. Nickell University of Kentucky, [email protected] Patrick Breheny University of Kentucky, [email protected] Arnold J. Stromberg University of Kentucky, [email protected] Timothy S. McClintock University of Kentucky, [email protected] Right click to open a feedback form in a new tab to let us know how this document benefits oy u. Follow this and additional works at: https://uknowledge.uky.edu/physiology_facpub Part of the Genomics Commons, and the Physiology Commons Repository Citation Nickell, Melissa D.; Breheny, Patrick; Stromberg, Arnold J.; and McClintock, Timothy S., "Genomics of Mature and Immature Olfactory Sensory Neurons" (2012). Physiology Faculty Publications. 66. https://uknowledge.uky.edu/physiology_facpub/66 This Article is brought to you for free and open access by the Physiology at UKnowledge. It has been accepted for inclusion in Physiology Faculty Publications by an authorized administrator of UKnowledge. For more information, please contact [email protected]. Genomics of Mature and Immature Olfactory Sensory Neurons Notes/Citation Information Published in Journal of Comparative Neurology, v. 520, issue 12, p. 2608-2629. Copyright © 2012 Wiley Periodicals, Inc. This is the peer reviewed version of the following article: Nickell, M. D., Breheny, P., Stromberg, A. J., and McClintock, T. S. (2012). Genomics of mature and immature olfactory sensory neurons. Journal of Comparative Neurology, 520: 2608–2629, which has been published in final form at http://dx.doi.org/ 10.1002/cne.23052. This article may be used for non-commercial purposes in accordance with Wiley Terms and Conditions for Self-Archiving. -
Agrin Controls Synaptic Differentiation in Hippocampal Neurons
The Journal of Neuroscience, December 15, 2000, 20(24):9086–9095 Agrin Controls Synaptic Differentiation in Hippocampal Neurons Christian M. Bo¨ se,1 Dike Qiu,1 Andrea Bergamaschi,3 Biagio Gravante,3 Mario Bossi,2 Antonello Villa,2 Fabio Rupp,1 and Antonio Malgaroli3 1Department of Neuroscience, The Johns Hopkins University, School of Medicine, Baltimore, Maryland 21205, 2Microscopy and Image Analysis, University of Milan School of Medicine, and 3Department of Biological and Technological Research, Scientific Institute San Raffaele, 20123 Milano, Italy Agrin controls the formation of the neuromuscular junction. agrin and could not be detected in cultures from agrin-deficient Whether it regulates the differentiation of other types of synapses animals. Interestingly, the few synapses formed in reduced agrin remains unclear. Therefore, we have studied the role of agrin in conditions displayed diminished vesicular turnover, despite a cultured hippocampal neurons. Synaptogenesis was severely normal appearance at the EM level. Thus, our results demon- compromised when agrin expression or function was sup- strate the necessity of agrin for synaptogenesis in hippocampal pressed by antisense oligonucleotides and specific antibodies. neurons. The effects of antisense oligonucleotides were found to be highly Key words: agrin; synaptogenesis; synapses; primary hip- specific because they were reversed by adding recombinant pocampal neurons; agrin antibodies; antisense oligonucleotides The fidelity of neural transmission depends on interactions of a can be detected in CNS neurons, where the temporal pattern of large number of presynaptic and postsynaptic molecules. Despite expression of agrin parallels synaptogenesis (Hoch et al., 1993; the importance of these processes for neural communication, the O’Connor et al., 1994; Stone and Nikolics, 1995; N.