Function and Regulation of Kinesin-1, -2 and -3
Total Page:16
File Type:pdf, Size:1020Kb
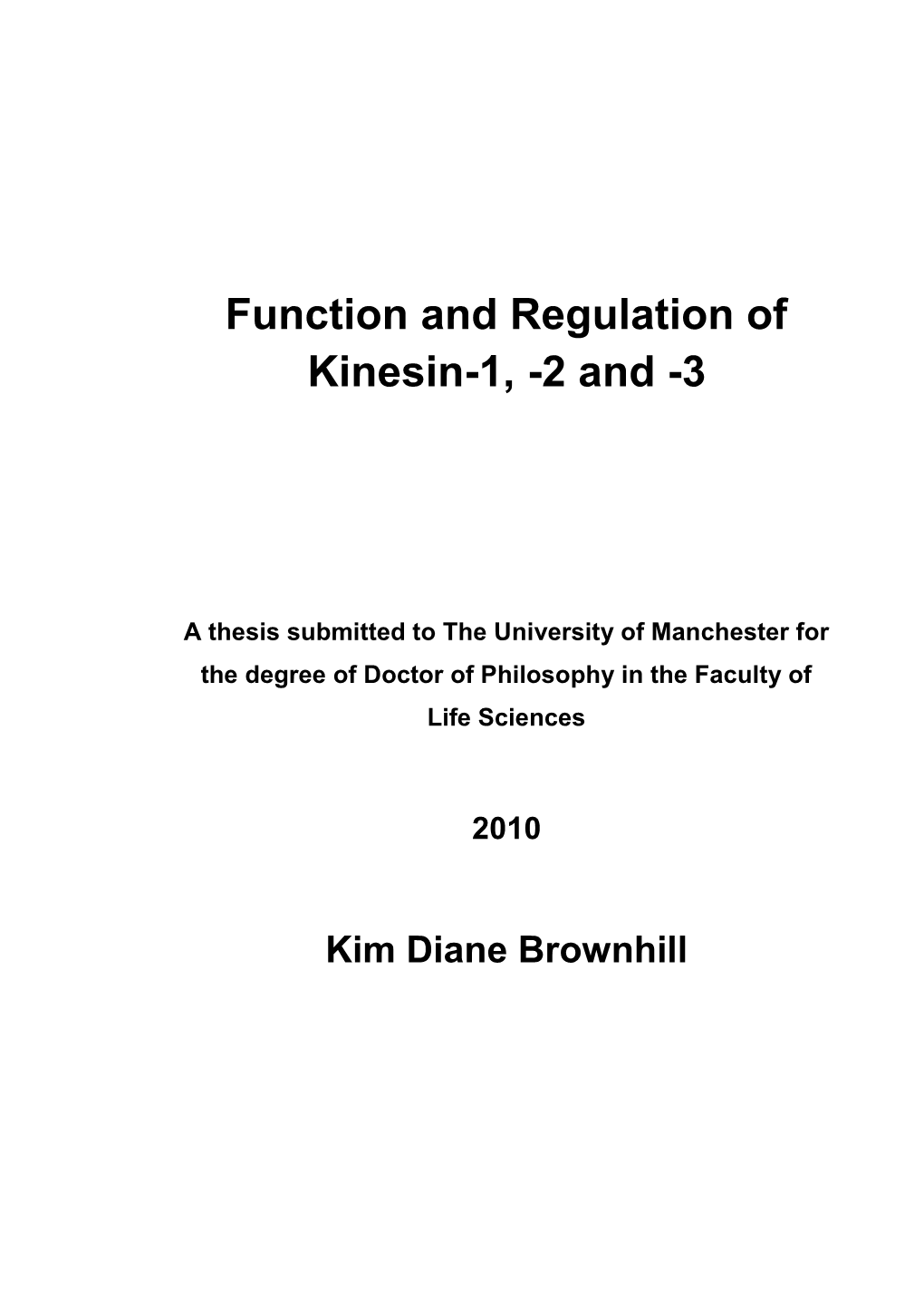
Load more
Recommended publications
-
Sorting Nexins in Protein Homeostasis Sara E. Hanley1,And Katrina F
Preprints (www.preprints.org) | NOT PEER-REVIEWED | Posted: 6 November 2020 doi:10.20944/preprints202011.0241.v1 Sorting nexins in protein homeostasis Sara E. Hanley1,and Katrina F. Cooper2* 1Department of Molecular Biology, Graduate School of Biomedical Sciences, Rowan University, Stratford, NJ, 08084, USA 1 [email protected] 2 [email protected] * [email protected] Tel: +1 (856)-566-2887 1Department of Molecular Biology, Graduate School of Biomedical Sciences, Rowan University, Stratford, NJ, 08084, USA Abstract: Sorting nexins (SNXs) are a highly conserved membrane-associated protein family that plays a role in regulating protein homeostasis. This family of proteins is unified by their characteristic phox (PX) phosphoinositides binding domain. Along with binding to membranes, this family of SNXs also comprises a diverse array of protein-protein interaction motifs that are required for cellular sorting and protein trafficking. SNXs play a role in maintaining the integrity of the proteome which is essential for regulating multiple fundamental processes such as cell cycle progression, transcription, metabolism, and stress response. To tightly regulate these processes proteins must be expressed and degraded in the correct location and at the correct time. The cell employs several proteolysis mechanisms to ensure that proteins are selectively degraded at the appropriate spatiotemporal conditions. SNXs play a role in ubiquitin-mediated protein homeostasis at multiple levels including cargo localization, recycling, degradation, and function. In this review, we will discuss the role of SNXs in three different protein homeostasis systems: endocytosis lysosomal, the ubiquitin-proteasomal, and the autophagy-lysosomal system. The highly conserved nature of this protein family by beginning with the early research on SNXs and protein trafficking in yeast and lead into their important roles in mammalian systems. -
Structural and Functional Insights Into Sorting Nexin 5/6 Interaction with Bacterial Effector Ince
OPEN Citation: Signal Transduction and Targeted Therapy (2017) 2, e17030; doi:10.1038/sigtrans.2017.30 www.nature.com/sigtrans ARTICLE Structural and functional insights into sorting nexin 5/6 interaction with bacterial effector IncE Qingxiang Sun1,5, Xin Yong1,2,5, Xiaodong Sun3,5, Fan Yang1,2,5, Zhonghua Dai4, Yanqiu Gong1, Liming Zhou3, Xia Zhang1, Dawen Niu1, Lunzhi Dai1, Jia-Jia Liu4 and Da Jia1,2 The endosomal trafficking pathways are essential for many cellular activities. They are also important targets by many intracellular pathogens. Key regulators of the endosomal trafficking include the retromer complex and sorting nexins (SNXs). Chlamydia trachomatis effector protein IncE directly targets the retromer components SNX5 and SNX6 and suppresses retromer-mediated transport, but the exact mechanism has remained unclear. We present the crystal structure of the PX domain of SNX5 in complex with IncE, showing that IncE binds to a highly conserved hydrophobic groove of SNX5. The unique helical hairpin of SNX5/6 is essential for binding, explaining the specificity of SNX5/6 for IncE. The SNX5/6–IncE interaction is required for cellular localization of IncE and its inhibitory function. Mechanistically, IncE inhibits the association of CI-MPR cargo with retromer-containing endosomal subdomains. Our study provides new insights into the regulation of retromer-mediated transport and illustrates the intricate competition between host and pathogens in controlling cellular trafficking. Signal Transduction and Targeted Therapy (2017) 2, e17030; doi:10.1038/sigtrans.2017.30; -
A Computational Approach for Defining a Signature of Β-Cell Golgi Stress in Diabetes Mellitus
Page 1 of 781 Diabetes A Computational Approach for Defining a Signature of β-Cell Golgi Stress in Diabetes Mellitus Robert N. Bone1,6,7, Olufunmilola Oyebamiji2, Sayali Talware2, Sharmila Selvaraj2, Preethi Krishnan3,6, Farooq Syed1,6,7, Huanmei Wu2, Carmella Evans-Molina 1,3,4,5,6,7,8* Departments of 1Pediatrics, 3Medicine, 4Anatomy, Cell Biology & Physiology, 5Biochemistry & Molecular Biology, the 6Center for Diabetes & Metabolic Diseases, and the 7Herman B. Wells Center for Pediatric Research, Indiana University School of Medicine, Indianapolis, IN 46202; 2Department of BioHealth Informatics, Indiana University-Purdue University Indianapolis, Indianapolis, IN, 46202; 8Roudebush VA Medical Center, Indianapolis, IN 46202. *Corresponding Author(s): Carmella Evans-Molina, MD, PhD ([email protected]) Indiana University School of Medicine, 635 Barnhill Drive, MS 2031A, Indianapolis, IN 46202, Telephone: (317) 274-4145, Fax (317) 274-4107 Running Title: Golgi Stress Response in Diabetes Word Count: 4358 Number of Figures: 6 Keywords: Golgi apparatus stress, Islets, β cell, Type 1 diabetes, Type 2 diabetes 1 Diabetes Publish Ahead of Print, published online August 20, 2020 Diabetes Page 2 of 781 ABSTRACT The Golgi apparatus (GA) is an important site of insulin processing and granule maturation, but whether GA organelle dysfunction and GA stress are present in the diabetic β-cell has not been tested. We utilized an informatics-based approach to develop a transcriptional signature of β-cell GA stress using existing RNA sequencing and microarray datasets generated using human islets from donors with diabetes and islets where type 1(T1D) and type 2 diabetes (T2D) had been modeled ex vivo. To narrow our results to GA-specific genes, we applied a filter set of 1,030 genes accepted as GA associated. -
Endosome‑ER Contacts Control Actin Nucleation and Retromer Function Through VAP‑Dependent Regulation of PI4P
This document is downloaded from DR‑NTU (https://dr.ntu.edu.sg) Nanyang Technological University, Singapore. Endosome‑ER Contacts Control Actin Nucleation and Retromer Function through VAP‑Dependent Regulation of PI4P Dong, Rui; Saheki, Yasunori; Swarup, Sharan; Lucast, Louise; Harper, J. Wade; De Camilli, Pietro 2016 Dong, R., Saheki, Y., Swarup, S., Lucast, L., Harper, J., & De Camilli, P. (2016). Endosome‑ER Contacts Control Actin Nucleation and Retromer Function through VAP‑Dependent Regulation of PI4P. Cell, 166(2), 408‑423. https://hdl.handle.net/10356/83386 https://doi.org/10.1016/j.cell.2016.06.037 © 2016 Elsevier Inc. This is the author created version of a work that has been peer reviewed and accepted for publication by Cell, Elsevier Inc. It incorporates referee’s comments but changes resulting from the publishing process, such as copyediting, structural formatting, may not be reflected in this document. The published version is available at: [http://dx.doi.org/10.1016/j.cell.2016.06.037]. Downloaded on 08 Oct 2021 15:56:00 SGT Endosome-ER contacts control actin nucleation and retromer function through VAP-dependent regulation of PI4P Rui Dong1, 2, 3, 5, Yasunori Saheki1, 2, 3, 5, 7, Sharan Swarup6, Louise Lucast1, 2, 3, 5, J. Wade Harper6, Pietro De Camilli1, 2, 3, 4, 5, *. 1Department of Neuroscience, Yale University School of Medicine, New Haven, Connecticut 06510, USA. 2Department of Cell Biology, Yale University School of Medicine, New Haven, CT 06510, USA. 3Howard Hughes Medical Institute, Yale University School of Medicine, New Haven, CT 06510, USA. 4Kavli Institute for Neurosciences, Yale University School of Medicine, New Haven, CT 06510, USA. -
Molecular Mechanism for the Subversion of the Retromer Coat By
Molecular mechanism for the subversion of the PNAS PLUS retromer coat by the Legionella effector RidL Miguel Romano-Morenoa, Adriana L. Rojasa, Chad D. Williamsonb, David C. Gershlickb, María Lucasa, Michail N. Isupovc, Juan S. Bonifacinob, Matthias P. Machnerd,1, and Aitor Hierroa,e,1 aStructural Biology Unit, Centro de Investigación Cooperativa en Biociencias, 48160 Derio, Spain; bCell Biology and Neurobiology Branch, Eunice Kennedy Shriver National Institute of Child Health and Human Development, National Institutes of Health, Bethesda, MD 20892; cThe Henry Wellcome Building for Biocatalysis, Biosciences, University of Exeter, Exeter EX4 4SB, United Kingdom; dDivision of Molecular and Cellular Biology, Eunice Kennedy Shriver National Institute of Child Health and Human Development, National Institutes of Health, Bethesda, MD 20892; and eIKERBASQUE, Basque Foundation for Science, 48011 Bilbao, Spain Edited by Ralph R. Isberg, Howard Hughes Medical Institute and Tufts University School of Medicine, Boston, MA, and approved November 13, 2017 (received for review August 30, 2017) Microbial pathogens employ sophisticated virulence strategies to VAMP7 together with several Rab GTPases that function along cause infections in humans. The intracellular pathogen Legionella distinct trafficking pathways (18), and the Tre-2/Bub2/Cdc16 pneumophila encodes RidL to hijack the host scaffold protein domain family member 5 (TBC1D5), a GTPase-activating pro- VPS29, a component of retromer and retriever complexes critical for tein (GAP) that causes Rab7 inactivation and redistribution to endosomal cargo recycling. Here, we determined the crystal structure the cytosol (14). of L. pneumophila RidL in complex with the human VPS29–VPS35 Recent biochemical and structural characterization of single retromer subcomplex. A hairpin loop protruding from RidL inserts subunits and subcomplexes from retromer have provided insights into a conserved pocket on VPS29 that is also used by cellular ligands, into its modular architecture and mechanisms of action. -
Table S1. Identified Proteins with Exclusive Expression in Cerebellum of Rats of Control, 10Mg F/L and 50Mg F/L Groups
Table S1. Identified proteins with exclusive expression in cerebellum of rats of control, 10mg F/L and 50mg F/L groups. Accession PLGS Protein Name Group IDa Score Q3TXS7 26S proteasome non-ATPase regulatory subunit 1 435 Control Q9CQX8 28S ribosomal protein S36_ mitochondrial 197 Control P52760 2-iminobutanoate/2-iminopropanoate deaminase 315 Control Q60597 2-oxoglutarate dehydrogenase_ mitochondrial 67 Control P24815 3 beta-hydroxysteroid dehydrogenase/Delta 5-->4-isomerase type 1 84 Control Q99L13 3-hydroxyisobutyrate dehydrogenase_ mitochondrial 114 Control P61922 4-aminobutyrate aminotransferase_ mitochondrial 470 Control P10852 4F2 cell-surface antigen heavy chain 220 Control Q8K010 5-oxoprolinase 197 Control P47955 60S acidic ribosomal protein P1 190 Control P70266 6-phosphofructo-2-kinase/fructose-2_6-bisphosphatase 1 113 Control Q8QZT1 Acetyl-CoA acetyltransferase_ mitochondrial 402 Control Q9R0Y5 Adenylate kinase isoenzyme 1 623 Control Q80TS3 Adhesion G protein-coupled receptor L3 59 Control B7ZCC9 Adhesion G-protein coupled receptor G4 139 Control Q6P5E6 ADP-ribosylation factor-binding protein GGA2 45 Control E9Q394 A-kinase anchor protein 13 60 Control Q80Y20 Alkylated DNA repair protein alkB homolog 8 111 Control P07758 Alpha-1-antitrypsin 1-1 78 Control P22599 Alpha-1-antitrypsin 1-2 78 Control Q00896 Alpha-1-antitrypsin 1-3 78 Control Q00897 Alpha-1-antitrypsin 1-4 78 Control P57780 Alpha-actinin-4 58 Control Q9QYC0 Alpha-adducin 270 Control Q9DB05 Alpha-soluble NSF attachment protein 156 Control Q6PAM1 Alpha-taxilin 161 -
Kinesin-2 Controls the Motility of RAB5 Endosomes and Their Association with the Spindle in Mitosis
International Journal of Molecular Sciences Article Kinesin-2 Controls the Motility of RAB5 Endosomes and Their Association with the Spindle in Mitosis Emanuela Pupo 1,2,*, Daniele Avanzato 1,2, Marco Scianna 3, Amanda Oldani 4, Guido Serini 1,2 and Letizia Lanzetti 1,2,* ID 1 Department of Oncology, University of Torino Medical School, 10060 Torino, Italy; [email protected] (D.A.); [email protected] (G.S.) 2 Candiolo Cancer Institute, FPO–IRCCS, Candiolo, 10060 Torino, Italy 3 Department of Mathematical Sciences, Politecnico di Torino, 10129 Torino, Italy; [email protected] 4 IFOM, The FIRC Institute for Molecular Oncology Foundation, 20139 Milan, Italy; [email protected] * Correspondence: [email protected] (E.P.); [email protected] (L.L.); Tel.: +39-011-993-3240 (E.P.); +39-011-993-3255 (L.L.); Fax: +39-011-993-3524 (L.L.) Received: 8 August 2018; Accepted: 27 August 2018; Published: 30 August 2018 Abstract: RAB5 is a small GTPase that belongs to the wide family of Rab proteins and localizes on early endosomes. In its active GTP-bound form, RAB5 recruits downstream effectors that, in turn, are responsible for distinct aspects of early endosome function, including their movement along microtubules. We previously reported that, at the onset of mitosis, RAB5positive vesicles cluster around the spindle poles and, during metaphase, move along spindle microtubules. RNAi-mediated depletion of the three RAB5 isoforms delays nuclear envelope breakdown at prophase and severely affects chromosome alignment and segregation. Here we show that depletion of the Kinesin-2 motor complex impairs long-range movement of RAB5 endosomes in interphase cells and prevents localization of these vesicles at the spindle during metaphase. -