Recent Progress in Flexible Wearable Sensors for Vital Sign Monitoring
Total Page:16
File Type:pdf, Size:1020Kb
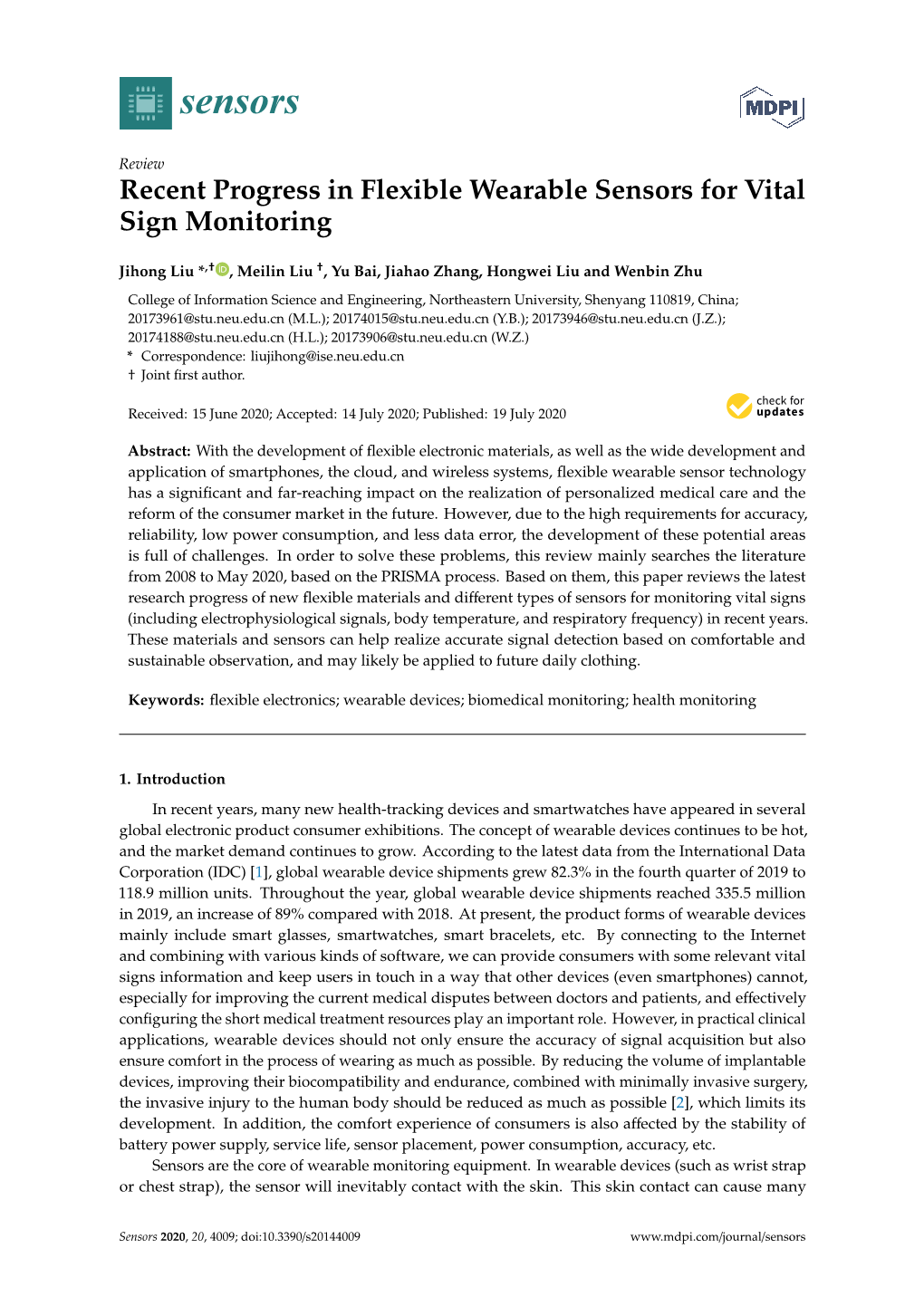
Load more
Recommended publications
-
Arxiv:1811.01044V2 [Physics.Med-Ph] 23 Jan 2019 Potentially Be Extended to Include Variability Among Individuals
Cardiovascular function and ballistocardiogram: a relationship interpreted via mathematical modeling Giovanna Guidoboni1, Lorenzo Sala2, Moein Enayati3, Riccardo Sacco4, Marcela Szopos5, James Keller3, Mihail Popescu6, Laurel Despins7, Virginia H. Huxley8, and Marjorie Skubic3 1 Department of Electrical Engineering and Computer Science and with the Department of Mathematics, University of Missouri, Columbia, MO, 65211 USA email: [email protected]. 2Universit´ede Strasbourg, CNRS, IRMA UMR 7501, Strasbourg, France. 5Universit´eParis Descartes, MAP5, UMR CNRS 8145, Paris, France. 3Department of Electrical Engineering and Computer Science, University of Missouri, Columbia, MO, 65211 USA. 4Dipartimento di Matematica, Politecnico di Milano, Piazza Leonardo da Vinci 32, 20133 Milano, Italy. 6Department of Health Management and Informatics, University of Missouri, Columbia, MO, 65211 USA. 7Sinclair School of Nursing, University of Missouri, Columbia, MO, 65211 USA. 8Department of Medical Pharmacology and Physiology, University of Missouri, Columbia, MO, 65211 USA. Abstract Objective: to develop quantitative methods for the clinical interpretation of the ballistocardiogram (BCG). Methods: a closed-loop mathematical model of the cardiovascular system is proposed to theoretically simulate the mechanisms generating the BCG signal, which is then compared with the signal acquired via accelerometry on a suspended bed. Results: simulated arterial pressure waveforms and ventricular functions are in good qualitative and quantitative agreement with those reported in the clinical literature. Simulated BCG signals exhibit the typical I, J, K, L, M and N peaks and show good qualitative and quantitative agreement with experimental measurements. Simulated BCG signals associated with reduced contractility and increased stiffness of the left ventricle exhibit different changes that are characteristic of the specific pathological con- dition. -
Influence of Sympathetic Activation on Myocardial Contractility Measured 4 with Ballistocardiography and Seismocardiography During Sustained End- 5 Expiratory Apnea
Ballistocardiography, seismocardiography and sympathetic nerve activity 1 TITLE PAGE 2 3 Influence of sympathetic activation on myocardial contractility measured 4 with ballistocardiography and seismocardiography during sustained end- 5 expiratory apnea. 6 Ballistocardiography, seismocardiography and sympathetic nerve activity 7 8 9 Sofia Morra, MD1, Anais Gauthey MD2, Amin Hossein MSc3, Jérémy Rabineau MSc3, Judith 10 Racape, PhD5, Damien Gorlier MSc3, Pierre-François Migeotte, MSc, PhD3, Jean Benoit le Polain de 11 Waroux, MD, PhD4, Philippe van de Borne MD, PhD1 12 13 14 1Department of Cardiology, Erasme hospital, Université Libre de Bruxelles, Belgium 15 2 Department of Cardiology, Saint-Luc hospital, Université Catholique de Louvain, Belgium 16 3LPHYS, Université Libre de Bruxelles, Belgium 17 4 Department of Cardiology, Sint-Jan, Hospital Bruges, Bruges, Belgium 18 5 Research centre in epidemiology, biostatistics and clinical research. School of Public Health. Université libre 19 de Bruxelles (ULB), Brussels, Belgium 20 21 22 23 24 25 26 27 28 29 30 31 32 33 34 35 36 37 38 39 40 1 Downloaded from journals.physiology.org/journal/ajpregu at Cornell Univ (132.174.252.179) on September 7, 2020. Ballistocardiography, seismocardiography and sympathetic nerve activity 41 42 43 44 45 46 47 NOTE AND NOTEWORTHY 48 49 50 Ballistocardiography (BCG) and seismocardiography (SCG) assess vibrations produced by cardiac 51 contraction and blood flow, respectively, through micro-accelerometers and micro-gyroscopes. 52 Kinetic energies (KE), and their temporal integrals (iK) during a single heartbeat are computed 53 from the BCG and SCG waveforms in a linear and a rotational dimension. When compared to 54 normal breathing, during an end-expiratory voluntary apnea, iK increased and was positively 55 related to sympathetic nerve traffic rise assessed by microneurography. -
Chapter 2 Ballistocardiography
POLITECNICO DI TORINO Corso di Laurea Magistrale in Ingegneria Biomedica Tesi di Laurea Magistrale Ballistocardiographic heart and breathing rates detection Relatore: Candidato: Prof.ssa Gabriella Olmo Emanuela Stirparo ANNO ACCADEMICO 2018-2019 Acknowledgements A conclusione di questo lavoro di tesi vorrei ringraziare tutte le persone che mi hanno sostenuta ed accompagnata lungo questo percorso. Ringrazio la Professoressa Gabriella Olmo per avermi dato la possibilità di svolgere la tesi nell’azienda STMicroelectronics e per la disponibilità mostratami. Ringrazio l’intero team: Luigi, Stefano e in particolare Marco, Valeria ed Alessandro per avermi aiutato in questo percorso con suggerimenti e consigli. Grazie per essere sempre stati gentili e disponibili, sia in campo professionale che umano. Un ringraziamento lo devo anche a Giorgio, per avermi fornito tutte le informazioni e i dettagli tecnici in merito al sensore utilizzato. Vorrei ringraziare anche tutti i ragazzi che hanno condiviso con me questa esperienza ed hanno contribuito ad alleggerire le giornate lavorative in azienda. Ringrazio i miei amici e compagni di università: Ilaria e Maria, le amiche sulle quali posso sempre contare nonostante la distanza; Rocco, compagno di viaggio, per i consigli e per aver condiviso con me gioie così come l’ansia e le paure per gli esami; Valentina e Beatrice perché ci sono e ci sono sempre state; Rosy, compagna di studi ma anche di svago; Sara, collega diligente e sempre con una parola di supporto, grazie soprattuto per tutte le dritte di questo ultimo periodo. Il più grande ringraziamento va ai miei genitori, il mio punto di riferimento, il mio sostegno di questi anni. -
Bed-Based Ballistocardiography: Dataset and Ability to Track Cardiovascular Parameters
sensors Article Bed-Based Ballistocardiography: Dataset and Ability to Track Cardiovascular Parameters Charles Carlson 1,* , Vanessa-Rose Turpin 2, Ahmad Suliman 1 , Carl Ade 2, Steve Warren 1 and David E. Thompson 1 1 Mike Wiegers Department of Electrical and Computer Engineering, Kansas State University, Manhattan, KS 66506, USA; [email protected] (A.S.); [email protected] (S.W.); [email protected] (D.E.T.) 2 Department of Kinesiology, Kansas State University, Manhattan, KS 66506, USA; [email protected] (V.-R.T.); [email protected] (C.A.) * Correspondence: [email protected] Abstract: Background: The goal of this work was to create a sharable dataset of heart-driven signals, including ballistocardiograms (BCGs) and time-aligned electrocardiograms (ECGs), photoplethysmo- grams (PPGs), and blood pressure waveforms. Methods: A custom, bed-based ballistocardiographic system is described in detail. Affiliated cardiopulmonary signals are acquired using a GE Datex CardioCap 5 patient monitor (which collects ECG and PPG data) and a Finapres Medical Systems Finometer PRO (which provides continuous reconstructed brachial artery pressure waveforms and derived cardiovascular parameters). Results: Data were collected from 40 participants, 4 of whom had been or were currently diagnosed with a heart condition at the time they enrolled in the study. An investigation revealed that features extracted from a BCG could be used to track changes in systolic blood pressure (Pearson correlation coefficient of 0.54 +/− 0.15), dP/dtmax (Pearson correlation coefficient of 0.51 +/− 0.18), and stroke volume (Pearson correlation coefficient of 0.54 +/− 0.17). Conclusion: A collection of synchronized, heart-driven signals, including BCGs, ECGs, PPGs, and blood pressure waveforms, was acquired and made publicly available. -
Assessment of Trends in the Cardiovascular System from Time Interval Measurements Using Physiological Signals Obtained at the Limbs
ADVERTIMENT . La consulta d’aquesta tesi queda condicionada a l’acceptació de les següents condicions d'ús: La difusió d’aquesta tesi per mitjà del servei TDX ( www.tesisenxarxa.net ) ha estat autoritzada pels titulars dels drets de propietat intel·lectual únicament per a usos privats emmarcats en activitats d’investigació i docència. No s’autoritza la seva reproducció amb finalitats de lucre ni la seva difusió i posada a disposició des d’un lloc aliè al servei TDX. No s’autoritza la presentació del seu contingut en una finestra o marc aliè a TDX (framing). Aquesta reserva de drets afecta tant al resum de presentació de la tesi com als seus continguts. En la utilització o cita de parts de la tesi és obligat indicar el nom de la persona autora. ADVERTENCIA . La consulta de esta tesis queda condicionada a la aceptación de las siguientes condiciones de uso: La difusión de esta tesis por medio del servicio TDR ( www.tesisenred.net ) ha sido autorizada por los titulares de los derechos de propiedad intelectual únicamente para usos privados enmarcados en actividades de investigación y docencia. No se autoriza su reproducción con finalidades de lucro ni su difusión y puesta a disposición desde un sitio ajeno al servicio TDR. No se autoriza la presentación de su contenido en una ventana o marco ajeno a TDR (framing). Esta reserva de derechos afecta tanto al resumen de presentación de la tesis como a sus contenidos. En la utilización o cita de partes de la tesis es obligado indicar el nombre de la persona autora. -
Printable Version [PDF]
CPC - A61B - 2021.08 A61B DIAGNOSIS; SURGERY; IDENTIFICATION (analysing biological material G01N, e.g. G01N 33/48; obtaining records using waves other than optical waves, in general G03B 42/00) Definition statement This place covers: Assessment or monitoring based on detecting, measuring or recording of or related to the prosthesis, e.g. measuring motion or position of prosthesis or measurement of physiological parameters or signals, such as myoelectric signals. The detecting, measuring or recording means may or may not be located on or in the prosthesis. The measurement, detection or recording may for example be used as an input signal useful for the control of prosthesis. • internal or external portions of the bodies (e.g., lungs), • abnormal bodily conditions (e.g., sickness, broken bones, detecting foreign bodies, pregnancy), • mental conditions (e.g., psychotechniques), and • bodily functions (e.g., heart beat, vision). Apparatus, instruments, implements, or processes that are either specially adapted or intended to be solely utilized for medical procedures employing physical actions (e.g., laser cutting, pressure of fluid) on portions of human or animal bodies to correct, enhance, or inspect (e.g., autopsies) them for medical purposes (i.e., surgery). Surgery consists of the following medical procedures: • repositioning (e.g. aligning broken bones, opening wounds) parts of bodies, • stabilizing (e.g., inserting bone pins) to prevent harmful movement of parts of bodies, • repairing (e.g., fastening skin together, removing cancerous tissue) bodies, • facilitating the occurrence of naturally occurring bodily functions (e.g., child birth, passing kidney stones) that are out of the ordinary, • introducing, collecting, or removing cells and organs (e.g., inseminations, tissue sampling, hair transplants, skin grafting, biopsies, organ harvesting) to or from bodies, and • introducing or taking out foreign objects (e.g., replacement heart valves, bullets) to or from bodies. -
Ballistocardiography Eduardo Pinheiro*, Octavian Postolache and Pedro Girão
The Open Biomedical Engineering Journal, 2010, 4, 201-216 201 Open Access Theory and Developments in an Unobtrusive Cardiovascular System Representation: Ballistocardiography Eduardo Pinheiro*, Octavian Postolache and Pedro Girão Instituto de Telecomunicações, Instituto Superior Técnico, Torre Norte piso 10, Av. Rovisco Pais 1, 1049-001, Lisboa, Portugal Abstract: Due to recent technological improvements, namely in the field of piezoelectric sensors, ballistocardiography – an almost forgotten physiological measurement – is now being object of a renewed scientific interest. Transcending the initial purposes of its development, ballistocardiography has revealed itself to be a useful informative signal about the cardiovascular system status, since it is a non-intrusive technique which is able to assess the body’s vibrations due to its cardiac, and respiratory physiological signatures. Apart from representing the outcome of the electrical stimulus to the myocardium – which may be obtained by electrocardiography – the ballistocardiograph has additional advantages, as it can be embedded in objects of common use, such as a bed or a chair. Moreover, it enables measurements without the presence of medical staff, factor which avoids the stress caused by medical examinations and reduces the patient’s involuntary psychophysiological responses. Given these attributes, and the crescent number of systems developed in recent years, it is therefore pertinent to revise all the information available on the ballistocardiogram’s physiological interpretation, its typical waveform information, its features and distortions, as well as the state of the art in device implementations. Keywords: Ballistocardiography, biomedical measurements, cardiac signal analysis, cardiovascular system monitoring, unobtrusive instrumentation. INTRODUCTION golden age of ballistocardiography, were labelled: “high frequency” [8], “low frequency” [9], and “ultra-low Ballistocardiography (coined from the Greek, frequency” [4, 5, 10-14]. -
Cardiovascular Function and Ballistocardiogram
Cardiovascular function and ballistocardiogram: a relationship interpreted via mathematical modeling Giovanna Guidoboni, Lorenzo Sala, Moein Enayati, Riccardo Sacco, Marcela Szopos, James Keller, Mihail Popescu, Laurel Despins, Virginia Huxley, Marjorie Skubic To cite this version: Giovanna Guidoboni, Lorenzo Sala, Moein Enayati, Riccardo Sacco, Marcela Szopos, et al.. Car- diovascular function and ballistocardiogram: a relationship interpreted via mathematical modeling. IEEE Transactions on Biomedical Engineering, Institute of Electrical and Electronics Engineers, 2019, 10.1109/TBME.2019.2897952. hal-02278848 HAL Id: hal-02278848 https://hal.archives-ouvertes.fr/hal-02278848 Submitted on 4 Sep 2019 HAL is a multi-disciplinary open access L’archive ouverte pluridisciplinaire HAL, est archive for the deposit and dissemination of sci- destinée au dépôt et à la diffusion de documents entific research documents, whether they are pub- scientifiques de niveau recherche, publiés ou non, lished or not. The documents may come from émanant des établissements d’enseignement et de teaching and research institutions in France or recherche français ou étrangers, des laboratoires abroad, or from public or private research centers. publics ou privés. Cardiovascular function and ballistocardiogram: a relationship interpreted via mathematical modeling Giovanna Guidoboni1, Lorenzo Sala2, Moein Enayati3, Riccardo Sacco4, Marcela Szopos5, James Keller3, Mihail Popescu6, Laurel Despins7, Virginia H. Huxley8, and Marjorie Skubic3 1 Department of Electrical Engineering and Computer Science and with the Department of Mathematics, University of Missouri, Columbia, MO, 65211 USA email: [email protected]. 2Universit´ede Strasbourg, CNRS, IRMA UMR 7501, Strasbourg, France. 5Universit´eParis Descartes, MAP5, UMR CNRS 8145, Paris, France. 3Department of Electrical Engineering and Computer Science, University of Missouri, Columbia, MO, 65211 USA. -
Three-Dimensional Apex-Seismocardiography
Three-Dimensional Apex-Seismocardiography Samuel E Schmidt, Ask S Jensen, Jacob Melgaard, Claus Graff, John Hansen, Tanveer A Bhuiyan, Johannes J Struijk Department of Health Science and Technology Aalborg University, Aalborg, Denmark Abstract family of cardiac vibration quantification technologies like phonocardiography and Ballistocardiography, which Traditional apex-cardiography measures chest wall all had their golden age before the emergence of vibrations at the apex beat using an air-coupled echocardiography. However, modern electronics as microphone. To provide additional insight in apex- microprocessors, tablets and advanced signal cardiography and chest vibrations we estimated the three processing/classification might make these technologies dimensional displacement of the apex beat. very low cost and easy to use, which might spur new A 3-axis accelerometer was placed at the location of applications and revitalise these technologies [2-4]. the apex beat in 5 healthy subjects in left lateral Specific advantages of apex-cardiography compared to decubitus position. ECG, echo Doppler of the carotid phonocardiography include its relation to left ventricular artery and phonocardiography were recorded pressure and the possibility to identify the timing of aortic simultaneous. The 3D displacements of the apex beat and mitral valve openings [5-6]. Modern applications of were estimated by twofold integration of the apex-cardiography might include screening for heart accelerometer signal. The most dominating displacement failure or quantification of ventricular synchrony. direction was estimated as the largest eigen vector in a To provide additional insight in the apex beat and principal component analysis (PCA). apex-cardiography we estimated the three dimensional The peak-to-peak displacements in the longitudinal, displacement of the apex beat using a 3-axis transverse and perpendicular dimensions were 0.39±0.35 accelerometer. -
Cardiovascular Adaptation to Simulated Microgravity And
www.nature.com/scientificreports OPEN Cardiovascular adaptation to simulated microgravity and countermeasure efcacy assessed by ballistocardiography and seismocardiography Jeremy Rabineau1,2*, Amin Hossein1, Federica Landreani3, Benoit Haut2, Edwin Mulder4, Elena Luchitskaya5, Jens Tank4, Enrico G. Caiani3, Philippe van de Borne6 & Pierre‑François Migeotte1 Head‑down bed rest (HDBR) reproduces the cardiovascular efects of microgravity. We tested the hypothesis that regular high‑intensity physical exercise (JUMP) could prevent this cardiovascular deconditioning, which could be detected using seismocardiography (SCG) and ballistocardiography (BCG). 23 healthy males were exposed to 60‑day HDBR: 12 in a physical exercise group (JUMP), the others in a control group (CTRL). SCG and BCG were measured during supine controlled breathing protocols. From the linear and rotational SCG/BCG signals, the integral of kinetic energy ( iK ) was computed on each dimension over the cardiac cycle. At the end of HDBR, BCG rotational iK and SCG transversal iK decreased similarly for all participants (− 40% and − 44%, respectively, p < 0.05), and so did orthostatic tolerance (− 58%, p < 0.01). Resting heart rate decreased in JUMP (− 10%, p < 0.01), but not in CTRL. BCG linear iK decreased in CTRL (− 50%, p < 0.05), but not in JUMP. The changes in the systolic component of BCG linear iK were correlated to those in stroke volume and VO2 max (R = 0.44 and 0.47, respectively, p < 0.05). JUMP was less afected by cardiovascular deconditioning, which could be detected by BCG in agreement with standard markers of the cardiovascular condition. This shows the potential of BCG to easily monitor cardiac deconditioning. -
Dynamic Time Warping for Heartbeat Detection in Ballistocardiography Guillaume Cathelain, Bertrand Rivet, Sophie Achard, Jean Bergounioux, François Jouen
Dynamic Time Warping for Heartbeat Detection in Ballistocardiography Guillaume Cathelain, Bertrand Rivet, Sophie Achard, Jean Bergounioux, François Jouen To cite this version: Guillaume Cathelain, Bertrand Rivet, Sophie Achard, Jean Bergounioux, François Jouen. Dynamic Time Warping for Heartbeat Detection in Ballistocardiography. CinC 2019 - Computing in Cardiology Conference, Sep 2019, Singapour, Singapore. 10.22489/CinC.2019.145. hal-03078460 HAL Id: hal-03078460 https://hal.archives-ouvertes.fr/hal-03078460 Submitted on 16 Dec 2020 HAL is a multi-disciplinary open access L’archive ouverte pluridisciplinaire HAL, est archive for the deposit and dissemination of sci- destinée au dépôt et à la diffusion de documents entific research documents, whether they are pub- scientifiques de niveau recherche, publiés ou non, lished or not. The documents may come from émanant des établissements d’enseignement et de teaching and research institutions in France or recherche français ou étrangers, des laboratoires abroad, or from public or private research centers. publics ou privés. Dynamic Time Warping for Heartbeat Detection in Ballistocardiography Guillaume Cathelain1, Bertrand Rivet2, Sophie Achard2, Jean Bergounioux3, François Jouen1 1 CHArt Laboratory, Ecole Pratique des Hautes Etudes, PSL University, Paris, France 2 Université Grenoble Alpes, CNRS, Grenoble INP, GIPSA-lab, Grenoble, France 3 Pediatric Intensive Care Unit, Assistance Publique des Hôpitaux de Paris, Garches, France Abstract times during hospitalization. Consequently, a non- intrusive apparatus is necessary to detect children Monitoring vital signs of neonates can be harmful and heartbeats in a harmless way. lead to developmental troubles. Ballistocardiography, a Ballistocardiography is a non-intrusive monitoring contactless heart rate monitoring method, has the method for cardiac activity. -
On the Performance of Bed-Integrated Ballistocardiography in Long-Term Heart Rate Monitoring of Vascular Patients
On the Performance of Bed-Integrated Ballistocardiography in Long-Term Heart Rate Monitoring of Vascular Patients Christoph Hoog Antink1, Yen Mai2, Jukka Ranta3, Adrian Tarniceriu4, Christoph Bruser¨ 1, Steffen Leonhardt1, Niku Oksala2;5, Antti Vehkaoja2 1Medical Information Technology, Helmholtz-Institute for Biomedical Engineering, RWTH Aachen University, Aachen, Germany 2Faculty of Medicine and Health Technology, Tampere University, Finland 3Emfit Oy, Vaajakoski, Finland, 4PulseOn SA, Neuchatel, Switzerland 5Vascular and Interventional Radiology Centre, Tampere University Hospital, Tampere, Finland Abstract sparse or unsystematic, and is mainly performed visually or with intermittent measurements. Continuous monitor- The application of ballistocardiography (BCG) for un- ing of hospital patients would be beneficial in the detection obtrusive monitor of (beat-to-beat) heart rate in sleep- of deterioration of a patient’s condition, which, if achieved ing subjects has seen increased attention in recent years. early, could enable preventive measures. While most studies are performed on healthy volunteers, The BCG technique has been proven adequately accu- some studies have included subjects with sleep-related rate for heart rate variability measurement with healthy problems. Even so, little attention has been payed to pa- subjects and bed-integrated [1–3] as well as wearable seis- tients suffering from cardiovascular diseases. In addition, mocardiography (SCG) sensors [4]. Bruser¨ et al. achieved most studies are either limited to short laboratory mea- 0.61 % relative error E¯rel with 85 % coverage in beat-to- surements or overnight recordings of sleeping subjects. beat interval (BBI) estimation with eight healthy subjects. In this work, we present preliminary results on beat- Corresponding numbers in the study by Kortelainen et al.