DEEP INELASTIC SCATTERING J. Drees Fachbereich Physik
Total Page:16
File Type:pdf, Size:1020Kb
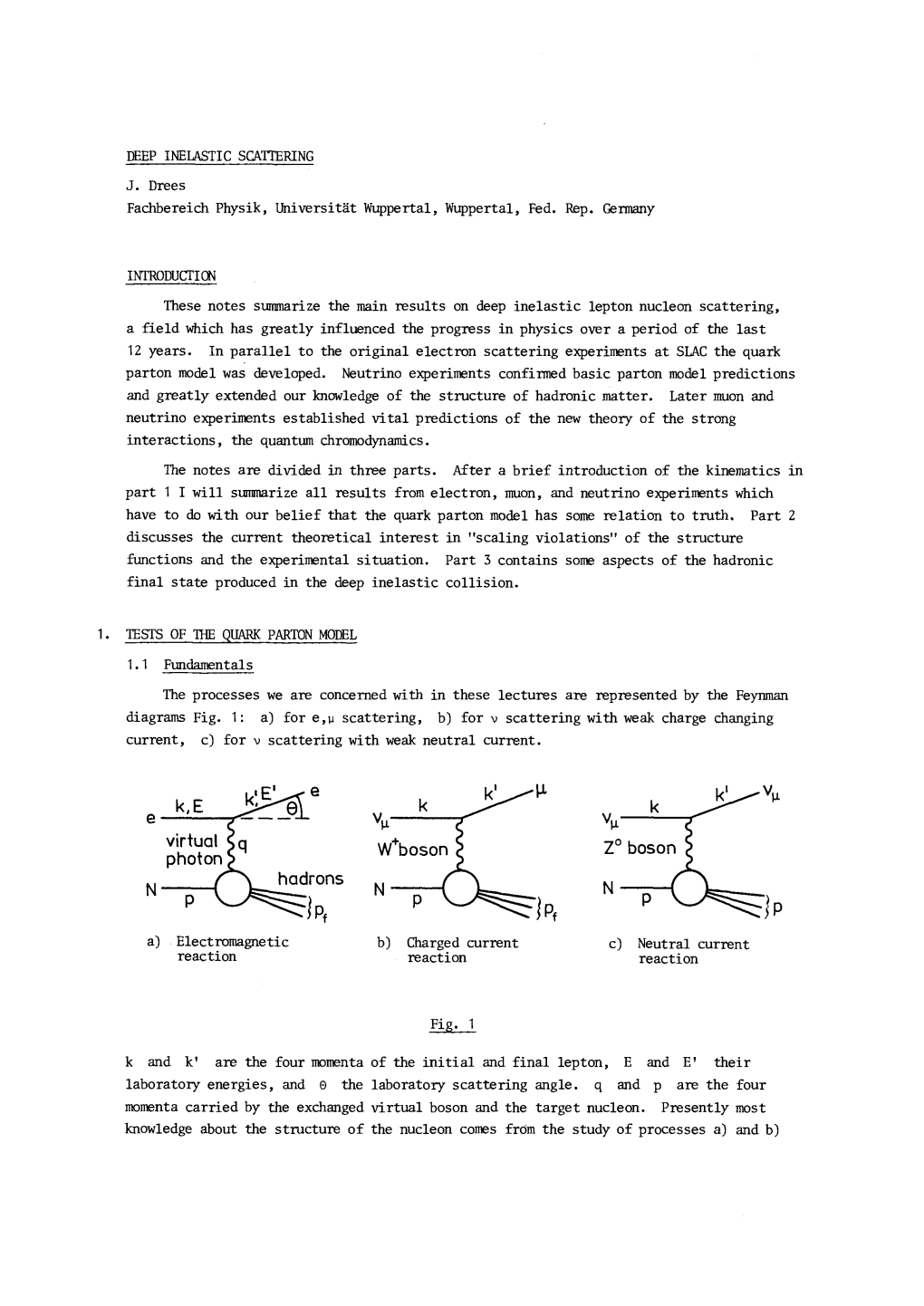
Load more
Recommended publications
-
Measurements of Elastic Electron-Proton Scattering at Large Momentum Transfer*
SLAC-PUB-4395 Rev. January 1993 m Measurements of Elastic Electron-Proton Scattering at Large Momentum Transfer* A. F. SILL,(~) R. G. ARNOLD,P. E. BOSTED,~. C. CHANG,@) J. GoMEz,(~)A. T. KATRAMATOU,C. J. MARTOFF, G. G. PETRATOS,(~)A. A. RAHBAR,S. E. ROCK,AND Z. M. SZALATA Department of Physics The American University, Washington DC 20016 D.J. SHERDEN Stanford Linear Accelerator Center , Stanford University, Stanford, California 94309 J. M. LAMBERT Department of Physics Georgetown University, Washington DC 20057 and R. M. LOMBARD-NELSEN De’partemente de Physique Nucle’aire CEN Saclay, Gif-sur- Yvette, 91191 Cedex, France Submitted to Physical Review D *Work supported by US Department of Energy contract DE-AC03-76SF00515 (SLAC), and National Science Foundation Grants PHY83-40337 and PHY85-10549 (American University). R. M. Lombard-Nelsen was supported by C. N. R. S. (French National Center for Scientific Research). Javier Gomez was partially supported by CONICIT, Venezuela. (‘)Present address: Department of Physics and Astronomy, University of Rochester, NY 14627. (*)Permanent address: Department of Physics and Astronomy, University of Maryland, College Park, MD 20742. (C)Present address: Continuous Electron Beam Accelerator Facility, Newport News, VA 23606. (d)Present address: Temple University, Philadelphia, PA 19122. (c)Present address: Stanford Linear Accelerator Center, Stanford CA 94309. ABSTRACT Measurements of the forward-angle differential cross section for elastic electron-proton scattering were made in the range of momentum transfer from Q2 = 2.9 to 31.3 (GeV/c)2 using an electron beam at the Stanford Linear Accel- erator Center. The data span six orders of magnitude in cross section. -
Impulse and Momentum
Impulse and Momentum All particles with mass experience the effects of impulse and momentum. Momentum and inertia are similar concepts that describe an objects motion, however inertia describes an objects resistance to change in its velocity, and momentum refers to the magnitude and direction of it's motion. Momentum is an important parameter to consider in many situations such as braking in a car or playing a game of billiards. An object can experience both linear momentum and angular momentum. The nature of linear momentum will be explored in this module. This section will discuss momentum and impulse and the interconnection between them. We will explore how energy lost in an impact is accounted for and the relationship of momentum to collisions between two bodies. This section aims to provide a better understanding of the fundamental concept of momentum. Understanding Momentum Any body that is in motion has momentum. A force acting on a body will change its momentum. The momentum of a particle is defined as the product of the mass multiplied by the velocity of the motion. Let the variable represent momentum. ... Eq. (1) The Principle of Momentum Recall Newton's second law of motion. ... Eq. (2) This can be rewritten with accelleration as the derivate of velocity with respect to time. ... Eq. (3) If this is integrated from time to ... Eq. (4) Moving the initial momentum to the other side of the equation yields ... Eq. (5) Here, the integral in the equation is the impulse of the system; it is the force acting on the mass over a period of time to . -
Part B. Radiation Sources Radiation Safety
Part B. Radiation sources Radiation Safety 1. Interaction of electrons-e with the matter −31 me = 9.11×10 kg ; E = m ec2 = 0.511 MeV; qe = -e 2. Interaction of photons-γ with the matter mγ = 0 kg ; E γ = 0 eV; q γ = 0 3. Interaction of neutrons-n with the matter −27 mn = 1.68 × 10 kg ; En = 939.57 MeV; qn = 0 4. Interaction of protons-p with the matter −27 mp = 1.67 × 10 kg ; Ep = 938.27 MeV; qp = +e Note: for any nucleus A: mass number – nucleons number A = Z + N Z: atomic number – proton (charge) number N: neutron number 1 / 35 1. Interaction of electrons with the matter Radiation Safety The physical processes: 1. Ionization losses inelastic collisions with orbital electrons 2. Bremsstrahlung losses inelastic collisions with atomic nuclei 3. Rutherford scattering elastic collisions with atomic nuclei Positrons at nearly rest energy: annihilation emission of two 511 keV photons 2 / 35 1. Interaction of electrons with1.E+02 the matter Radiation Safety ) 1.E+03 -1 Graphite – Z = 6 .g 2 1.E+01 ) Lead – Z = 82 -1 .g 2 1.E+02 1.E+00 1.E+01 1.E-01 1.E+00 collision 1.E-02 radiative collision 1.E-01 stopping pow er (MeV.cm total radiative 1.E-03 stopping pow er (MeV.cm total 1.E-02 1.E-01 1.E+00 1.E+01 1.E+02 1.E+03 1.E-02 energy (MeV) 1.E-02 1.E-01 1.E+00 1.E+01 1.E+02 1.E+03 Electrons – stopping power 1.E+02 energy (MeV) S 1 dE ) === -1 Copper – Z = 29 ρρρ ρρρ dl .g 2 1.E+01 S 1 dE 1 dE === +++ ρρρ ρρρ dl coll ρρρ dl rad 1 dE 1.E+00 : mass stopping power (MeV.cm 2 g. -
Neutron Scattering
Neutron Scattering Basic properties of neutron and electron neutron electron −27 −31 mass mkn =×1.675 10 g mke =×9.109 10 g charge 0 e spin s = ½ s = ½ −e= −e= magnetic dipole moment µnn= gs with gn = 3.826 µee= gs with ge = 2.0 2mn 2me =22k 2π =22k Ek== E = 2m λ 2m energy n e 81.81 150.26 Em[]eV = 2 Ee[]V = 2 λ ⎣⎡Å⎦⎤ λ ⎣⎦⎡⎤Å interaction with matter: Coulomb interaction — 9 strong-force interaction 9 — magnetic dipole-dipole 9 9 interaction Several salient features are apparent from this table: – electrons are charged and experience strong, long-range Coulomb interactions in a solid. They therefore typically only penetrate a few atomic layers into the solid. Electron scattering is therefore a surface-sensitive probe. Neutrons are uncharged and do not experience Coulomb interaction. The strong-force interaction is naturally strong but very short-range, and the magnetic interaction is long-range but weak. Neutrons therefore penetrate deeply into most materials, so that neutron scattering is a bulk probe. – Electrons with wavelengths comparable to interatomic distances (λ ~2Å ) have energies of several tens of electron volts, comparable to energies of plasmons and interband transitions in solids. Electron scattering is therefore well suited as a probe of these high-energy excitations. Neutrons with λ ~2Å have energies of several tens of meV , comparable to the thermal energies kTB at room temperature. These so-called “thermal neutrons” are excellent probes of low-energy excitations such as lattice vibrations and spin waves with energies in the meV range. -
Compton Sources of Electromagnetic Radiation∗
August 25, 2010 13:33 WSPC/INSTRUCTION FILE RAST˙Compton Reviews of Accelerator Science and Technology Vol. 1 (2008) 1–16 c World Scientific Publishing Company COMPTON SOURCES OF ELECTROMAGNETIC RADIATION∗ GEOFFREY KRAFFT Center for Advanced Studies of Accelerators, Jefferson Laboratory, 12050 Jefferson Ave. Newport News, Virginia 23606, United States of America kraff[email protected] GERD PRIEBE Division Leader High Field Laboratory, Max-Born-Institute, Max-Born-Straße 2 A Berlin, 12489, Germany [email protected] When a relativistic electron beam interacts with a high-field laser beam, the beam electrons can radiate intense and highly collimated electromagnetic radiation through Compton scattering. Through relativistic upshifting and the relativistic Doppler effect, highly energetic polarized photons are radiated along the electron beam motion when the electrons interact with the laser light. For example, X-ray radiation can be obtained when optical lasers are scattered from electrons of tens of MeV beam energy. Because of the desirable properties of the radiation produced, many groups around the world have been designing, building, and utilizing Compton sources for a wide variety of purposes. In this review paper, we discuss the generation and properties of the scattered radiation, the types of Compton source devices that have been constructed to present, and the future prospects of radiation sources of this general type. Due to the possibilities to produce hard electromagnetic radiation in a device small compared to the alternative storage ring sources, it is foreseen that large numbers of such sources may be constructed in the future. Keywords: Compton backscattering, Inverse Compton source, Thomson scattering, X-rays, Spectral brilliance 1. -
AIAA 19Th Fluid Dynamics, Plasma Dynamics and Lasers Conference June 8-10, 1987/Honolulu, Hawaii
AIAA-87 -1407 Electron-Cyclotron-Resonance (ECR) Plasma Acceleration J. C. Sercel Jet Propulsion Laboratory California Institute of Technology Pasadena, California AIAA 19th Fluid Dynamics, Plasma Dynamics and Lasers Conference June 8-10, 1987/Honolulu, Hawaii For permission to copy or republish, contact the American Institute of Aeronautics and Astronautics 1633 Broadway, New York, NY 10019 AIAA-87-1407 ELECTRON-CYCLOTRON-RESONANCE (ECR) PLASMA ACCELERATION Joel C. Sercel* Jet Propulsion Laboratory California Institute of Technology Pasadena, California Abstract P power per unit volume, W/m3 R position vector, m A research effort directed at analytically v velocity, mls and experimentally investigating Electron U energy, J or eV Cyclotron-Resonance (ECR) plasma acceleration V electrostatic potential, volts is outlined. Relevant past research is reviewed. T temperature, Kelvin or eV The prospects for application of ECR plasma acceleration to spacecraft propulsion are described. It is shown that previously unexplained losses in converting microwave magnetic dipole moment power to directed kinetic power via ECR plasma reaction cross section, m2 acceleration can be understood in terms of diffusion of energized plasma to the physical time constant, s walls of the accelerator. It is argued that line radiation losses from electron-ion and electron SubscriPts atom inelastic collisions should be less than estimated in past research. Based on this new A acceleration understanding, the expectation now exists that B Bohm efficient ECR plasma accelerators can be e electron designed for application to high specific impulse ex excitation spacecraft propulsion. ionization summation variable refers to lowest energy level Acronyms and Abbreviations p perpendicular r relative D-He3 Deuterium Helium-Three sp space charge induced ECR Electron-Cyclotron-Resonance to t total GE General Electric JPL Jet Propulsion Laboratory LeRC Lewis Research Center I. -
The Basic Interactions Between Photons and Charged Particles With
Outline Chapter 6 The Basic Interactions between • Photon interactions Photons and Charged Particles – Photoelectric effect – Compton scattering with Matter – Pair productions Radiation Dosimetry I – Coherent scattering • Charged particle interactions – Stopping power and range Text: H.E Johns and J.R. Cunningham, The – Bremsstrahlung interaction th physics of radiology, 4 ed. – Bragg peak http://www.utoledo.edu/med/depts/radther Photon interactions Photoelectric effect • Collision between a photon and an • With energy deposition atom results in ejection of a bound – Photoelectric effect electron – Compton scattering • The photon disappears and is replaced by an electron ejected from the atom • No energy deposition in classical Thomson treatment with kinetic energy KE = hν − Eb – Pair production (above the threshold of 1.02 MeV) • Highest probability if the photon – Photo-nuclear interactions for higher energies energy is just above the binding energy (above 10 MeV) of the electron (absorption edge) • Additional energy may be deposited • Without energy deposition locally by Auger electrons and/or – Coherent scattering Photoelectric mass attenuation coefficients fluorescence photons of lead and soft tissue as a function of photon energy. K and L-absorption edges are shown for lead Thomson scattering Photoelectric effect (classical treatment) • Electron tends to be ejected • Elastic scattering of photon (EM wave) on free electron o at 90 for low energy • Electron is accelerated by EM wave and radiates a wave photons, and approaching • No -
Particles and Deep Inelastic Scattering
Heidi Schellman Northwestern Particles and Deep Inelastic Scattering Heidi Schellman Northwestern University HUGS - JLab - June 2010 June 2010 HUGS 1 Heidi Schellman Northwestern k’ k q P P’ A generic scatter of a lepton off of some target. kµ and k0µ are the 4-momenta of the lepton and P µ and P 0µ indicate the target and the final state of the target, which may consist of many particles. qµ = kµ − k0µ is the 4-momentum transfer to the target. June 2010 HUGS 2 Heidi Schellman Northwestern Lorentz invariants k’ k q P P’ 2 2 02 2 2 2 02 2 2 2 The 5 invariant masses k = m` , k = m`0, P = M , P ≡ W , q ≡ −Q are invariants. In addition you can define 3 Mandelstam variables: s = (k + P )2, t = (k − k0)2 and u = (P − k0)2. 2 2 2 2 s + t + u = m` + M + m`0 + W . There are also handy variables ν = (p · q)=M , x = Q2=2Mµ and y = (p · q)=(p · k). June 2010 HUGS 3 Heidi Schellman Northwestern In the lab frame k’ k θ q M P’ The beam k is going in the z direction. Confine the scatter to the x − z plane. µ k = (Ek; 0; 0; k) P µ = (M; 0; 0; 0) 0µ 0 0 0 k = (Ek; k sin θ; 0; k cos θ) qµ = kµ − k0µ June 2010 HUGS 4 Heidi Schellman Northwestern In the lab frame k’ k θ q M P’ 2 2 2 s = ECM = 2EkM + M − m ! 2EkM 2 0 0 2 02 0 t = −Q = −2EkEk + 2kk cos θ + mk + mk ! −2kk (1 − cos θ) 0 ν = (p · q)=M = Ek − Ek energy transfer to target 0 y = (p · q)=(p · k) = (Ek − Ek)=Ek the inelasticity P 02 = W 2 = 2Mν + M 2 − Q2 invariant mass of P 0µ June 2010 HUGS 5 Heidi Schellman Northwestern In the CM frame k’ k q P P’ The beam k is going in the z direction. -
Analysis of Thruster Requirements and Capabilities for Local Satellite Clusters
I I ANALYSIS OF THRUSTER REQUIREMENTS AND CAPABILITIES FOR LOCAL SATELLITE CLUSTERS G. I. Yashkot, D.E. Hastingstt I Massachusetts Institute of Technology Cambridge, Massachusetts I Abstract This paper examines the propulsive requirements necessary to maintain the relative positions of satellites orbiting in a local cluster. Formation of these large baseline arrays could allow high resolution imaging of terrestrial or I astronomical targets using techniques similar to those used for decades in radio interferometry. A key factor in the image quality is the relative positions of the individual apertures in the sparse array. The relative positions of satellites in a cluster are altered by "tidal" accelerations which are a function of the cluster baseline and orbit altitude. These accelerations must be counteracted by continuous thrusting to maintain the relative positions of the satellites. I Analysis of propulsive system requirements, limited by spacecraft power, volume, and mass constraints, indicates that specific impulses and efficiencies typical of ion engines or Hall thrusters (SPT's) are necessary to maintain large cluster baselines. In addition, required thrust to spacecraft mass ratios for reasonable size clusters are approximately I 15J..LNlkg. Finally, the ability of a proposed linear ion microthruster to meet these requirements is examined. A variation of Brophy's method is used to show that primary electron containment lengths on the order of 10 mm are I necessary to achieve those thruster characteristics. Preliminary sizing -
Chemical Applications of Inelastic X-Ray Scattering
BNL- 68406 CHEMICAL APPLICATIONS OF INELASTIC X-RAY SCATTERING H. Hayashi and Y. Udagawa Research Institute for Scientific Measurements Tohoku University Sendai, 980-8577, Japan J.-M. Gillet Structures, Proprietes et Modelisation des Solides UMR8580 Ecole Centrale Paris, Grande Voie des Vignes, 92295 Chatenay-Malabry Cedex, France W.A. Caliebe and C.-C. Kao National Synchrotron Light Source Brookhaven National Laboratory Upton, New York, 11973-5000, USA August 2001 National Synchrotron Light Source Brookhaven National Laboratory Operated by Brookhaven Science Associates Upton, NY 11973 Under Contract with the United States Department of Energy Contract Number DE-AC02-98CH10886 DISCLAIMER This report was prepared as an account of work sponsored by an agency of the United States Government. Neither the United States Government nor any agency thereof, nor any of their employees, nor any of their contractors, subcontractors or their employees, makes any warranty, express or implied, or assumes any legal liability or responsibility for the accuracy; completeness, or any third party’s use or the results of such use of any information, apparatus, product, or process disclosed, or represents that its use would not infringe privately owned rights. Reference herein to any specific commercial product, process, or service by trade name, trademark, manufacturer, or otherwise, does not necessarily constitute or imply its endorsement, recommendation, or favoring by the United States Government or any agency thereof or its contractors or subcontractors. Th.e views and opinions of authors expressed herein do not necessarily state or reflect those of the United States Government or any agency thereof. CHEMICAL APPLICATIONS OF INELASTIC X-RAY SCATTERING H. -
RCED-85-96 DOE's Physics Accelerators
DOE’s Physics Accelerators: Their Costs And Benefits This report provides an inventory of the Department of Energy’s existing and planned high-energy physics and nuclear physics accelerator facilities, identifies their as- sociated costs, and presents information on the benefits being derived from their construction and operation. These facilities are the primary tools used by high-energy and nuclear physicists to learn more about what energy and matter consist of and how their component parts or particles are influenced by the most basic natural forces. Of DOE’s $728 million budget for high-energy physics and nuclear physics during fiscal year 1985, about $372.1 million is earmarked for operating 14 DOE-supported accelerator facilities coast-to-coast. DOE’s investment in these facilities amounts to about $1.2 billion. If DOE’s current plans for adding new facilities are carried out, this investment could grow by about $4.3 billion through fiscal year 1994. Annual facility operating costs wilt also grow by about $230 million, or an increase of about 60 percent over current costs. The primary benefits gained from DOE’s investment in these facilities are new scientific knowledge and the education and training of future physicists. Accor- ding to DOE and accelerator facility officials, accelerator particle beams are also used in other scientific applications and have some medical and industrial applications. GAOIRCED-85-96 B APRIL 1,198s 031763 UNITED STATES GENERAL ACCOUNTING OFFICE WASHINGTON, D.C. 20548 RESOURCES, COMMUNlfY, \ND ECONOMIC DEVELOPMENT DIV ISlON B-217863 The Honorable J. Bennett Johnston Ranking Minority Member Subcommittee on Energy and Water Development Committee on Appropriations United States Senate Dear Senator Johnston: This report responds to your request dated November 25, 1984. -
Relative Merits and Limiting Factors for X-Ray and Electron Microscopy of Thick, Hydrated Organic Materials (2020 Revised Version)
Relative merits and limiting factors for x-ray and electron microscopy of thick, hydrated organic materials (2020 revised version) Ming Du1, and Chris Jacobsen2;3;4;∗ 1Department of Materials Science and Engineering, Northwestern University, 2145 Sheridan Road, Evanston IL 60208, USA 2Advanced Photon Source, Argonne National Laboratory, 9700 South Cass Avenue, Argonne IL 60439, USA 3Department of Physics & Astronomy, Northwestern University, 2145 Sheridan Road, Evanston IL 60208, USA 4Chemistry of Life Processes Institute, Northwestern University, 2170 Campus Drive, Evanston IL 60208, USA ∗Corresponding author; Email: [email protected] Abstract Electron and x-ray microscopes allow one to image the entire, unlabeled structure of hydrated mate- rials at a resolution well beyond what visible light microscopes can achieve. However, both approaches involve ionizing radiation, so that radiation damage must be considered as one of the limits to imaging. Drawing upon earlier work, we describe here a unified approach to estimating the image contrast (and thus the required exposure and corresponding radiation dose) in both x-ray and electron microscopy. This approach accounts for factors such as plural and inelastic scattering, and (in electron microscopy) the use of energy filters to obtain so-called \zero loss" images. As expected, it shows that electron microscopy offers lower dose for specimens thinner than about 1 µm (such as for studies of macromolecules, viruses, bacteria and archaebacteria, and thin sectioned material), while x-ray microscopy offers superior charac- teristics for imaging thicker specimen such as whole eukaryotic cells, thick-sectioned tissues, and organs. The required radiation dose scales strongly as a function of the desired spatial resolution, allowing one to understand the limits of live and frozen hydrated specimen imaging.