On Atmospheric Lapse Rates
Total Page:16
File Type:pdf, Size:1020Kb
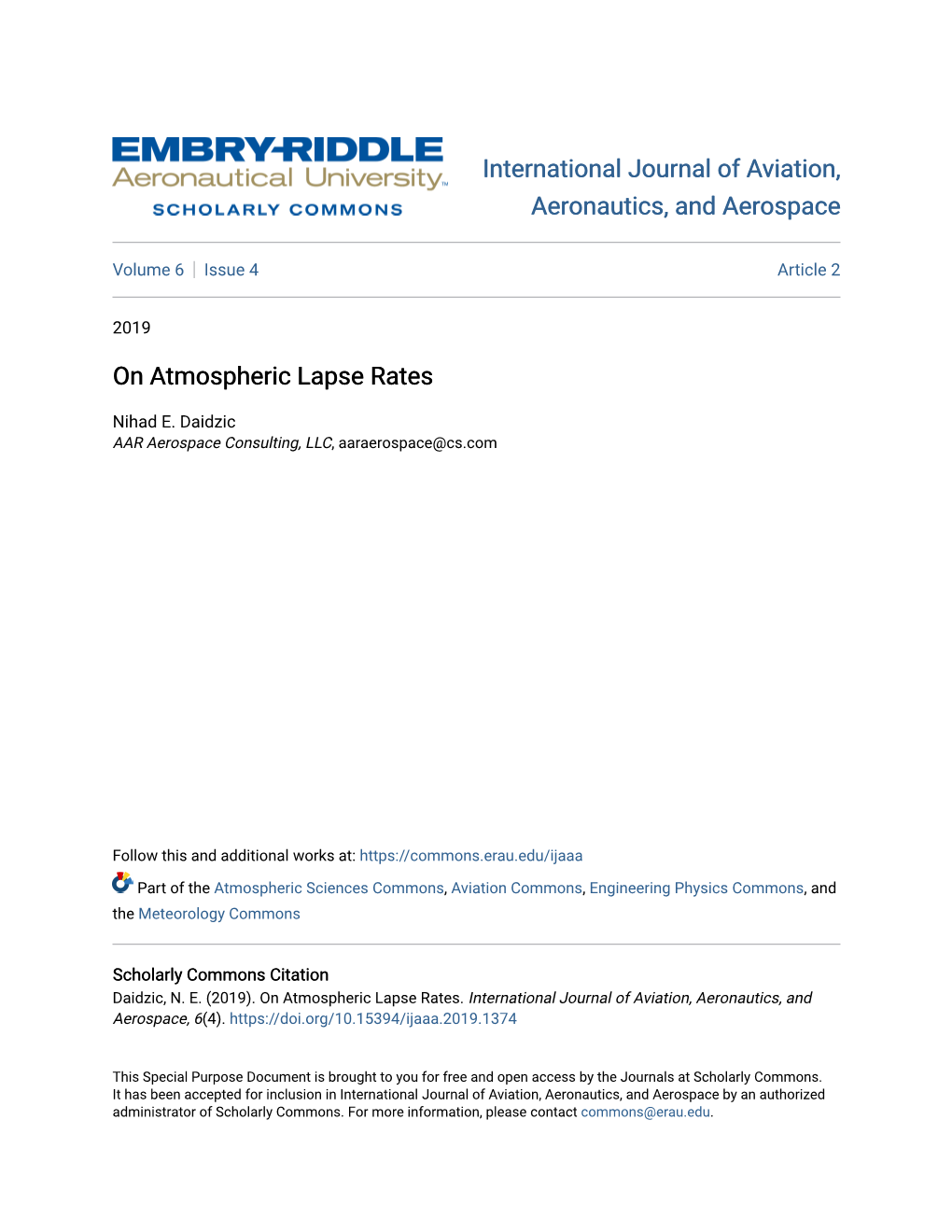
Load more
Recommended publications
-
Soaring Weather
Chapter 16 SOARING WEATHER While horse racing may be the "Sport of Kings," of the craft depends on the weather and the skill soaring may be considered the "King of Sports." of the pilot. Forward thrust comes from gliding Soaring bears the relationship to flying that sailing downward relative to the air the same as thrust bears to power boating. Soaring has made notable is developed in a power-off glide by a conven contributions to meteorology. For example, soar tional aircraft. Therefore, to gain or maintain ing pilots have probed thunderstorms and moun altitude, the soaring pilot must rely on upward tain waves with findings that have made flying motion of the air. safer for all pilots. However, soaring is primarily To a sailplane pilot, "lift" means the rate of recreational. climb he can achieve in an up-current, while "sink" A sailplane must have auxiliary power to be denotes his rate of descent in a downdraft or in come airborne such as a winch, a ground tow, or neutral air. "Zero sink" means that upward cur a tow by a powered aircraft. Once the sailcraft is rents are just strong enough to enable him to hold airborne and the tow cable released, performance altitude but not to climb. Sailplanes are highly 171 r efficient machines; a sink rate of a mere 2 feet per second. There is no point in trying to soar until second provides an airspeed of about 40 knots, and weather conditions favor vertical speeds greater a sink rate of 6 feet per second gives an airspeed than the minimum sink rate of the aircraft. -
Impact of Air Pollution Controls on Radiation Fog Frequency in the Central Valley of California
RESEARCH ARTICLE Impact of Air Pollution Controls on Radiation Fog 10.1029/2018JD029419 Frequency in the Central Valley of California Special Section: Ellyn Gray1 , S. Gilardoni2 , Dennis Baldocchi1 , Brian C. McDonald3,4 , Fog: Atmosphere, biosphere, 2 1,5 land, and ocean interactions Maria Cristina Facchini , and Allen H. Goldstein 1Department of Environmental Science, Policy and Management, Division of Ecosystem Sciences, University of 2 Key Points: California, Berkeley, CA, USA, Institute of Atmospheric Sciences and Climate ‐ National Research Council (ISAC‐CNR), • Central Valley fog frequency Bologna, BO, Italy, 3Cooperative Institute for Research in Environmental Science, University of Colorado, Boulder, CO, increased 85% from 1930 to 1970, USA, 4Chemical Sciences Division, NOAA Earth System Research Laboratory, Boulder, CO, USA, 5Department of Civil then declined 76% in the last 36 and Environmental Engineering, University of California, Berkeley, CA, USA winters, with large short‐term variability • Short‐term fog variability is dominantly driven by climate Abstract In California's Central Valley, tule fog frequency increased 85% from 1930 to 1970, then fluctuations declined 76% in the last 36 winters. Throughout these changes, fog frequency exhibited a consistent • ‐ Long term temporal and spatial north‐south trend, with maxima in southern latitudes. We analyzed seven decades of meteorological data trends in fog are dominantly driven by changes in air pollution and five decades of air pollution data to determine the most likely drivers changing fog, including temperature, dew point depression, precipitation, wind speed, and NOx (oxides of nitrogen) concentration. Supporting Information: Climate variables, most critically dew point depression, strongly influence the short‐term (annual) • Supporting Information S1 variability in fog frequency; however, the frequency of optimal conditions for fog formation show no • Table S1 • Table S2 observable trend from 1980 to 2016. -
Chapter 7: Stability and Cloud Development
Chapter 7: Stability and Cloud Development A parcel of air: Air inside can freely expand or contract, but heat and air molecules do not cross boundary. A rising parcel of air expands because the air pressure falls with elevation. This expansion causes cooling: (Adiabatic cooling) V V V-2v V v Wall Stationary Wall Moving Away An air molecule that strikes an expanding wall will lose speed on bouncing off wall (temperature of air will fall) V V+2v v Wall Moving Toward A falling parcel of air contracts because it encounters higher pressure. This falling air heats up because of compression. Stability: If rising air cools more quickly due to expansion than the environmental lapse rate, it will be denser than its surroundings and tend to fall back down. 10°C Definitions: Dry adiabatic rate = 1000 m 10°C Moist adiabatic rate : smaller than because of release of latent heat during 1000 m condensation (use 6° C per 1000m for average) Rising air that cools past dew point will not cool as quickly because of latent heat of condensation. Same compensating effect for saturated, falling air : evaporative cooling from water droplets evaporating reduces heating due to compression. Summary: Rising air expands & cools Falling air contracts & warms 10°C Unsaturated air cools as it rises at the dry adiabatic rate (~ ) 1000 m 6°C Saturated air cools at a lower rate, ~ , because condensing water vapor heats air. 1000 m This is moist adiabatic rate. Environmental lapse rate is rate that air temperature falls as you go up in altitude in the troposphere. -
Chapter 8 Atmospheric Statics and Stability
Chapter 8 Atmospheric Statics and Stability 1. The Hydrostatic Equation • HydroSTATIC – dw/dt = 0! • Represents the balance between the upward directed pressure gradient force and downward directed gravity. ρ = const within this slab dp A=1 dz Force balance p-dp ρ p g d z upward pressure gradient force = downward force by gravity • p=F/A. A=1 m2, so upward force on bottom of slab is p, downward force on top is p-dp, so net upward force is dp. • Weight due to gravity is F=mg=ρgdz • Force balance: dp/dz = -ρg 2. Geopotential • Like potential energy. It is the work done on a parcel of air (per unit mass, to raise that parcel from the ground to a height z. • dφ ≡ gdz, so • Geopotential height – used as vertical coordinate often in synoptic meteorology. ≡ φ( 2 • Z z)/go (where go is 9.81 m/s ). • Note: Since gravity decreases with height (only slightly in troposphere), geopotential height Z will be a little less than actual height z. 3. The Hypsometric Equation and Thickness • Combining the equation for geopotential height with the ρ hydrostatic equation and the equation of state p = Rd Tv, • Integrating and assuming a mean virtual temp (so it can be a constant and pulled outside the integral), we get the hypsometric equation: • For a given mean virtual temperature, this equation allows for calculation of the thickness of the layer between 2 given pressure levels. • For two given pressure levels, the thickness is lower when the virtual temperature is lower, (ie., denser air). • Since thickness is readily calculated from radiosonde measurements, it provides an excellent forecasting tool. -
The Gas Laws and the Weather
IMPACT 1 ON ENVIRONMENTAL SCIENCE The gas laws and the weather The biggest sample of gas readily accessible to us is the atmos- 24 phere, a mixture of gases with the composition summarized in Table 1. The composition is maintained moderately constant by diffusion and convection (winds, particularly the local turbu- 18 lence called eddies) but the pressure and temperature vary with /km altitude and with the local conditions, particularly in the tropo- h sphere (the ‘sphere of change’), the layer extending up to about 11 km. 12 In the troposphere the average temperature is 15 °C at sea level, falling to −57 °C at the bottom of the tropopause at 11 km. This Altitude, variation is much less pronounced when expressed on the Kelvin 6 scale, ranging from 288 K to 216 K, an average of 268 K. If we suppose that the temperature has its average value all the way up to the tropopause, then the pressure varies with altitude, h, 0 according to the barometric formula: 0 0.5 1 Pressure, p/p0 −h/H p = p0e Fig. 1 The variation of atmospheric pressure with altitude, as predicted by where p0 is the pressure at sea level and H is a constant approxi- the barometric formula and as suggested by the ‘US Standard Atmosphere’, mately equal to 8 km. More specifically,H = RT/Mg, where M which takes into account the variation of temperature with altitude. is the average molar mass of air and T is the temperature. This formula represents the outcome of the competition between the can therefore be associated with rising air and clear skies are potential energy of the molecules in the gravitational field of the often associated with descending air. -
Forecasting of Thunderstorms in the Pre-Monsoon Season at Delhi
View metadata, citation and similar papers at core.ac.uk brought to you by CORE provided by Publications of the IAS Fellows Meteorol. Appl. 6, 29–38 (1999) Forecasting of thunderstorms in the pre-monsoon season at Delhi N Ravi1, U C Mohanty1, O P Madan1 and R K Paliwal2 1Centre for Atmospheric Sciences, Indian Institute of Technology, New Delhi 110 016, India 2National Centre for Medium Range Weather Forecasting, Mausam Bhavan Complex, Lodi Road, New Delhi 110 003, India Accurate prediction of thunderstorms during the pre-monsoon season (April–June) in India is essential for human activities such as construction, aviation and agriculture. Two objective forecasting methods are developed using data from May and June for 1985–89. The developed methods are tested with independent data sets of the recent years, namely May and June for the years 1994 and 1995. The first method is based on a graphical technique. Fifteen different types of stability index are used in combinations of different pairs. It is found that Showalter index versus Totals total index and Jefferson’s modified index versus George index can cluster cases of occurrence of thunderstorms mixed with a few cases of non-occurrence along a zone. The zones are demarcated and further sub-zones are created for clarity. The probability of occurrence/non-occurrence of thunderstorms in each sub-zone is then calculated. The second approach uses a multiple regression method to predict the occurrence/non- occurrence of thunderstorms. A total of 274 potential predictors are subjected to stepwise screening and nine significant predictors are selected to formulate a multiple regression equation that gives the forecast in probabilistic terms. -
Global Warming Climate Records
ATM S 111: Global Warming Climate Records Jennifer Fletcher Day 24: July 26 2010 Reading For today: “Keeping Track” (Climate Records) pp. 171-192 For tomorrow/Wednesday: “The Long View” (Paleoclimate) pp. 193-226. The Instrumental Record from NASA Global temperature since 1880 Ten warmest years: 2001-2009 and 1998 (biggest El Niño ever) Separation into Northern/ Southern Hemispheres N. Hem. has warmed more (1o C vs 0.8o C globally) S. Hem. has warmed more steadily though Cooling in the record from 1940-1975 essentially only in the N. Hem. record (this is likely due to aerosol cooling) Temperature estimates from other groups CRUTEM3 (RG calls this UEA) NCDC (RG calls this NOAA) GISS (RG calls this NASA) yet another group Surface air temperature over land Thermometer between 1.25-2 m (4-6.5 ft) above ground White colored to reflect away direct sunlight Slats to ensure fresh air circulation “Stevenson screen”: invented by Robert Louis Stevenson’s dad Thomas Yearly average Central England temperature record (since 1659) Temperatures over Land Only NASA separates their analysis into land station data only (not including ship measurements) Warming in the station data record is larger than in the full record (1.1o C as opposed to 0.8o C) Sea surface temperature measurements Standard bucket Canvas bucket Insulated bucket (~1891) (pre WWII) (now) “Bucket” temperature: older style subject to evaporative cooling Starting around WWII: many temperature measurements taken from condenser intake pipe instead of from buckets. Typically 0.5 C warmer -
CHAPTER NO.4 Psychrometry
CHAPTER NO.4 Psychrometry C605.4-Explain Psychometric properties & calculate various parameters. Necessity of Air conditioning Purpose of air conditioning is 1) To provide comfort conditions for human comfort. 2) To provide comfort in commercial places like office, restaurant ,shopping complex , banks etc. 3) To provide controlled conditions for industrial process. 4) To provide ultra clean atmosphere for precision work. Dalton’s low of partial pressure • Dalton’s law of partial pressure statures that ‘the total pressure of mixture of gases equal to the sum of the partial pressures exerted by each gas when it occupies the mixture volume at there temperature of mixture’. • According to Dalton’s law of partial pressure, • Pt = Pa + Pb + Pc. Psychometrics properties of air 1. Dry air : It is a mixture of oxygen (20.91%) and nitrogen (79.09 %) by volume. 2. Moist air: It is mixture of dry air and water vapour. 3. Saturated air : Air which have maximum amount of water vapor. 4. Dry bulb temp.(DBT) : It is temperature of air recorded by ordinary thermometer with clean and dry sensing elements.(td or tdb) 5. Wet bulb temp.(WBT) : It is temperature of air recorded by thermometer when its bulb is covered with a wet cloth.(tw or twb) Psychometric properties of air 6. Wet bulb depression : It is the difference between dry bulb temp. and wet bulb temp. 7. Dew point temp.: It is the temperature of air recorded by thermometer when the moisture present in the air begins to condensed. 8. Dew point depression : It is difference between dry bulb temp. -
Atmospheric Stability Atmospheric Lapse Rate
ATMOSPHERIC STABILITY ATMOSPHERIC LAPSE RATE The atmospheric lapse rate ( ) refers to the change of an atmospheric variable with a change of altitude, the variable being temperature unless specified otherwise (such as pressure, density or humidity). While usually applied to Earth's atmosphere, the concept of lapse rate can be extended to atmospheres (if any) that exist on other planets. Lapse rates are usually expressed as the amount of temperature change associated with a specified amount of altitude change, such as 9.8 °Kelvin (K) per kilometer, 0.0098 °K per meter or the equivalent 5.4 °F per 1000 feet. If the atmospheric air cools with increasing altitude, the lapse rate may be expressed as a negative number. If the air heats with increasing altitude, the lapse rate may be expressed as a positive number. Understanding of lapse rates is important in micro-scale air pollution dispersion analysis, as well as urban noise pollution modeling, forest fire-fighting and certain aviation applications. The lapse rate is most often denoted by the Greek capital letter Gamma ( or Γ ) but not always. For example, the U.S. Standard Atmosphere uses L to denote lapse rates. A few others use the Greek lower case letter gamma ( ). Types of lapse rates There are three types of lapse rates that are used to express the rate of temperature change with a change in altitude, namely the dry adiabatic lapse rate, the wet adiabatic lapse rate and the environmental lapse rate. Dry adiabatic lapse rate Since the atmospheric pressure decreases with altitude, the volume of an air parcel expands as it rises. -
Atmospheric Thermal and Dynamic Vertical Structures of Summer Hourly Precipitation in Jiulong of the Tibetan Plateau
atmosphere Article Atmospheric Thermal and Dynamic Vertical Structures of Summer Hourly Precipitation in Jiulong of the Tibetan Plateau Yonglan Tang 1, Guirong Xu 1,*, Rong Wan 1, Xiaofang Wang 1, Junchao Wang 1 and Ping Li 2 1 Hubei Key Laboratory for Heavy Rain Monitoring and Warning Research, Institute of Heavy Rain, China Meteorological Administration, Wuhan 430205, China; [email protected] (Y.T.); [email protected] (R.W.); [email protected] (X.W.); [email protected] (J.W.) 2 Heavy Rain and Drought-Flood Disasters in Plateau and Basin Key Laboratory of Sichuan Province, Institute of Plateau Meteorology, China Meteorological Administration, Chengdu 610072, China; [email protected] * Correspondence: [email protected]; Tel.: +86-27-8180-4913 Abstract: It is an important to study atmospheric thermal and dynamic vertical structures over the Tibetan Plateau (TP) and their impact on precipitation by using long-term observation at represen- tative stations. This study exhibits the observational facts of summer precipitation variation on subdiurnal scale and its atmospheric thermal and dynamic vertical structures over the TP with hourly precipitation and intensive soundings in Jiulong during 2013–2020. It is found that precipitation amount and frequency are low in the daytime and high in the nighttime, and hourly precipitation greater than 1 mm mostly occurs at nighttime. Weak precipitation during the daytime may be caused by air advection, and strong precipitation at nighttime may be closely related with air convection. Both humidity and wind speed profiles show obvious fluctuation when precipitation occurs, and the greater the precipitation intensity, the larger the fluctuation. Moreover, the fluctuation of wind speed Citation: Tang, Y.; Xu, G.; Wan, R.; Wang, X.; Wang, J.; Li, P. -
THE INFRARED CLOUD IMAGER by Brentha Thurairajah a Thesis Submitted in Partial Fulfi
THERMAL INFRARED IMAGING OF THE ATMOSPHERE: THE INFRARED CLOUD IMAGER by Brentha Thurairajah A thesis submitted in partial fulfillment of the requirements of the degree of Master of Science in Electrical Engineering MONTANA STATE UNIVERSITY Bozeman, Montana April 2004 © COPYRIGHT by Brentha Thurairajah 2004 All Rights Reserved ii APPROVAL of a thesis submitted by Brentha Thurairajah This thesis has been read by each member of the thesis committee and has been found to be satisfactory regarding content, English usage, format, citations, bibliographic style, and consistency, and is ready for submission to the college of graduate studies. Dr. Joseph A. Shaw, Chair of Committee Approved for the Department of Electrical and Computer Engineering Dr. James N. Peterson, Department Head Approved for the College of Graduate Studies Dr. Bruce R. McLeod, Graduate Dean iii STATEMENT OF PERMISSION TO USE In presenting this thesis in partial fulfillment of the requirements for a master’s degree at Montana State University, I agree that the Library shall make it available to borrowers under the rules of the Library. If I have indicated my intention to copyright this thesis by including a copyright notice page, copying is allowable only for scholarly purposes, consistent with “fair use” as prescribed in the U.S Copyright Law. Requests for permission for extended quotation from or reproduction of this thesis in whole or in parts may be granted only by the copyright holder. Brentha Thurairajah April 2nd, 2004 iv ACKNOWLEDGEMENT I would like to thank my advisor Dr. Joseph Shaw for always being there, for guiding me with patience throughout the project, for helping me with my course and research work through all these three years, and for providing helpful comments and constructive criticism that helped me complete this document. -
Atmospheric Moisture
Name_____________________________________Date___________________Period________ Atmospheric Moisture Background Whether in solid, liquid or gaseous form, water is the most important component of the atmosphere and essentially helps control all other aspects of weather. However, in order to truly understand the atmosphere, simply describing moisture is not enough. In meteorology, several different moisture measurements are used to determine the overall stability and characteristics of the air. All of these “moisture variables” can be broken down into two categories. The first are those that are solely dependent upon the physical amount of water contained in the air and are known as absolute measurements. Relative measurements are those variables that not only depend upon the amount of water present but also the temperature of the air. Vapor Pressure (e) and Saturation Vapor Pressure (es) The total atmospheric pressure at any time is actually the sum of many small pressures for each of the atmosphere’s components. For example, oxygen, nitrogen, etc. all exert their own individual pressures that are all added together to create the overall atmospheric pressure. Vapor pressure is the portion of total pressure contributed by water. This amount is very small which is why huge differences in water vapor content in the atmosphere yield only small fluctuations in the overall atmospheric pressure. This measure is also an absolute measurement since it is solely dependent upon the actual amount of water vapor in the air. However, there is a maximum amount of pressure that water vapor can exert in the atmosphere before the water is squeezed out into a liquid again and condenses. This limit is known as the saturation vapor pressure and is dependent upon both water content and temperature.